Introduction
Pediatric neurosurgical procedures, for which intraoperative neuromonitoring (IONM) is utilized, can be divided into three broad categories: intracranial, extracranial decompression/stabilization, and extracranial mass lesion excisions. In adults IONM is frequently used for neurovascular procedures such as aneurysm clipping, arterial bypass procedures, and carotid endarterectomies. These procedures are uncommon in children; however, tumor resections, especially posterior fossa lesion resections, are far more frequently monitored in the pediatric population. These cases pose a number of challenges to the IONM team that increase with the size of the tumor and inversely with the age of the patient. Supratentorial lesions may pose risk to motor and sensory pathways directly, by virtue of their location, or indirectly by disturbing the vascular supply to motor/sensory pathways.
When IONM is used, it is important to understand the pathways that can be monitored and the limitations of the IONM data.1 For example, transcranial motor evoked potentials (TcMEPs) may be normal in a patient incapable of volitional movement due to injury to the supplemental motor area or the basal ganglia. A normal auditory brainstem response (ABR) is compatible with a preserved auditory pathway to the level of the inferior colliculus, but gives no information about the status of the primary auditory cortex. A full discussion of the limitation of IONM in the pediatric neurosurgical settings is beyond the scope of this chapter, but the major pathways monitored by each modality and the surgical procedures for which the modalities can provide useful information to the surgeon will be discussed. Prior to discussing the application of IONM to pediatric neurosurgical procedure, it is important to understand the combined impact of anesthesia and development on each of the IONM modalities that might be used during a given procedure.
The Impact of Anesthesia and Development of Specific Modalities
Signals that are recorded during IONM can be divided into two broad categories: (1) spontaneous activity such as cerebral electrical activity recorded as electroencephalography (EEG) and muscle activity that can be recorded as electromyography (EMG), and (2) evoked potentials. Evoked potentials are electrical responses to a specific stimulus. The response is typically recorded for a finite period of time after the stimulus; the relationship between stimulus and recording interval is known as time locking. Time locking can be used to advantage to reduce the admission of noise or to signal average multiple repetitions of a stimulus/recording trial, as is typically done for somatosensory evoked potentials and ABRs. In other cases such as TcMEPs the signals are sufficiently robust and have very high signal-to-noise ratios (SNR) that only a single trial is needed to obtain the desired data. Additionally, certain reflexes, such as the bulbocavernosus reflex, may be obtained on a single trial, if conditions are permissive.
Somatosensory Evoked Potentials
Somatosensory evoked potentials (SSEPs) are generated by repetitive stimulation of large peripheral nerves such as the ulnar, median, or posterior tibial nerve (PTN). The stimulus causes depolarization of 1 A and B fibers that carry proprioceptive, vibration, and light touch information.2 The nerve impulses ascend ipsilaterally in the dorsal columns to either the cuneate (upper extremities) or gracile (lower extremities and trunk) nuclei in the medulla. A cervical response can be recorded at the level of the fifth cervical vertebra as the impulses in the dorsal columns pass beneath the recording electrode. The presence of this potential provides an indication that the spinal cord pathways are intact to the C5 level. SSEP latencies to the C5 level will be less affected by marginal perfusion than motor evoked potentials because white matter is less metabolically active than gray matter. However, once the impulses that generate the component potentials of the SSEPs reach the intracranial level, both white and gray matter are involved and the effects of marginal perfusion are more easily detected by changes in latency and amplitude (Table 16.1).
Table 16.1 CNS Structures and Their Vascular Supplies Assessed by Median Nerve SSEPs
Structure | Vascular Supply | Potential Affected |
---|---|---|
Dorsal columns | Posterior spinal artery | N11 (P11) dorsal root entry zone |
Anterior serratus anterior (variant) | ||
Gracile/cuneate nucleus | Anterior spinal artery | N13 |
Derived from ventriculoatrial (VA) | ||
Medial lemniscus | Anterior spinal artery; vertebral arteries; paramedian branches of basilar artery; quadrageminal and medial posterior choroidal | P14 |
Thalamus–ventral posterolateral tier | Posterior choroidal artery | N18 |
Thalamocrtical projections | Lenticulostriates | |
Primary somatosensory cortex | M3 and M4 branches of MCA and A4 of ACA | N20, P25, P37 (PTN SSEPs) |
Notes:
(1)The vascular supplies listed derived from diagrams in Neuroanatomy: An Atlas of Structures, Sections, and Systems, D.E. Haines, 2008, Lippincott, Williams, and Wilkins.
(2)The listed vascular supplies are typical, but individual variation exists; for example, 30%, or more, of the total area of the dorsal columns has been shown to be supplied by branches of the anterior spinal artery in some individuals.
(3)The names assigned to the potentials are those assigned for adult patients. For patients younger than 8 years, N11 is N8, N14 is N9, and N20 is N16.
The next named potential is generated as the impulses reach the cuneate, or gracile, nucleus. If the impulses arrive at the brainstem nuclei in synchrony, the impulses are transmitted to the ventral-posterior lateral tiers of the thalamus via the arcuate fibers and medial lemniscus. As the impulses arrive at the thalamus, yet another potential is generated (P18). The impulses are relayed from the thalamus to the primary cortex. A potential known as the N20 marks the arrival of the impulses at the primary somatosensory cortex.3 The commonly named potentials for both a median nerve and PTN SSEPs are shown in Figures 16.1A and 16.1B, respectively. The peripheral potentials (usually recorded at Erb’s point), the cervical potential, and the median (ulnar) nerve cortical potentials are referred to as “near-field” potentials as the recording electrode is proximate to the site of generation. On the other hand the subcortical potential (N14–18) is a far-field potential, as the medial lemniscus is not near the recording sites. Far-field potentials are less sensitive to inexact placement of the recording electrodes than are near-field potentials.
Figures 16.1. Ulnar nerve (A) and posterior tibial nerve (B) SSEPs, respectively. (A) From the bottom to top, the montages represent the peripheral potentials recorded at Erb’s point, a cervical level response, the subcortical response, and the cortical response (top two). The short latencies of the Erb’s, cervical, and subcortical responses are due to the age of the patient. The Erb’s point to cortical interpeak latency is comparable to an adult indicating relative maturation of the central conduction pathways (see text). (B) The recording was obtained from a preteen undergoing a posterior spinal fusion. The bottom trace was recorded from electrodes in the popliteal fossa, the next trace up from the mastoid with a frontal reference, and the top three cortical montages provide data to determine the orientation of the dipole in 3-space.
The latencies of the various waves are affected by both anesthesia and postnatal development. Peripheral nerve conduction velocities increase with postnatal age and are not typically affected by anesthetic agents at clinical concentrations. Distances between stimulus site and recording site are much smaller than in an adult; consequently, peripheral (N9) and cervical potential (N13) latencies are much shorter in infants and toddlers than in adults. However, central conduction times are longer due to lack of myelination of central pathways. Myelination of the central pathways is generally complete by 2 years of age; the medial lemniscus is usually fully myelinated by 12 months and the thalamocortical projections by 12–18 months of age.4 Once the signals reach the medullary nuclei (gracile and cuneate), the effects of incomplete myelination manifest as a prolonged peripheral to cortical interpeak latency. Even in the absence of anesthesia, the amplitude of the cortical signals is attenuated compared to the adult due to dispersion of conduction velocities. The addition of anesthetic effects results in the near absence of recognizable cortical potentials in infants less than 1 month of age as SSEP latencies increase and amplitudes decrease in a dose-dependent manner with anesthetics. The only exception is etomidate, which increases the amplitude of evoked potentials compared to other agents.5 Median nerve SSEPs are typically recorded using a 100-µsec stimulus, applied at a rate of near 4 Hz in adults. When recording from infants, longer pulse lengths and lower stimulus frequencies must be used to record cortical signals on a consistent basis. This phenomenon can be partially overcome by using a lower stimulus frequency and longer pulse times.6, 7
Incomplete myelination in the spinal cord has a greater effect on the PTN SSEPs than on median nerve or ulnar nerve SSEPs because of the distance the impulses must travel within the spinal cord before reaching the medullary nuclei. Myelination of the dorsal columns proceeds in a cranial-caudal fashion and is complete at around 8 years of age. As a consequence of the delayed myelination of the dorsal columns, there tends to be greater dispersion of signals traveling on the dorsal columns of a 2-year-old than an adult resulting in a lower likelihood of generating a useable PTN cortical SSEP in the 2-year-old. Failure to obtain cortical PTN SSEPs in children less than 2 years of age is not uncommon;8 the rate of failure increases in the face of pathology within the spinal cord and with anesthesia/sedation. As in the case of the median nerve SSEPs, alteration of stimulus parameters is necessary when attempting to record PTN SSEPs from very young children.
SSEPs can also be used to locate the central sulcus when it is necessary to directly stimulate the primary motor cortex. A 1 × 4 grid can be placed on the brain while the median nerve on the contralateral wrist is stimulated. An upright N20 from a grid location indicates that the point is over the sensory cortex, while an inverted N20 indicates the grid point is over the motor cortex. Care must be taken to ensure that a transition from an upright to inverted N20 is clearly present in order to properly identify the central sulcus.
Transcranial Motor Evoked Potentials (TcMEPs)
Intraoperative and postoperative motor deficits can occur in the absence of changes in the SSEPs. Therefore, it is necessary to monitor the functional integrity of the entire motor pathway independently of the sensory tracts. Transcranial (Tc) and direct cortical (dc) motor evoked potentials (MEPs) serve this function (Table 16.2). TcMEPs are elicited by applying a high-voltage, short-duration stimulus to the scalp overlying the primary motor cortex.9 This electrical stimulus directly depolarizes the axons on the pyramidal neurons generating (D-waves) and activates cortical interneuronal networks in the motor cortex generating I-waves. The D-waves may initiate one of three or four locations within the intracranial portion of the corticospinal tract (CST), while the I-waves always originate from the cortex.10 Even in adults, lower extremity TcMEPs are more sensitive to anesthetic effects than upper extremity TcMEPs.11 Both D- and I-waves are conducted along the CST to depolarize a population of spinal alpha motor neurons (αMNs). The common teaching has been that D-waves are relatively resistant to the effects of anesthesia, but D-wave latency has been demonstrated to increase in a dose-dependent fashion when isoflurane is used for anesthesia. The generation of I-waves is inhibited by anesthetics, volatile agents having a greater effect than propofol; thus multipulse techniques are used to elicit TcMEPs under anesthesia.
Table 16.2 CNS Structures and Their Vascular Supplies Assessed by MEPs
Structure | Vascular Supply |
---|---|
Primary motor cortex | M3 and M4 branches of MCA and A4 of ACA |
Corona radiata internal capsule | Lenticulostriate arteries |
Anterior choroidal artery | |
Intracranial CST | Quadrageminal and posterior choroidal |
Basilar artery | |
Vertebral artery | |
Pyramidal decussation | Anterior spinal artery |
Derived from VA | |
Intraspinal CST | Anterior spinal artery (SC level) |
Alpha motor neurons | Anterior spinal artery (SC level) |
Notes:
(1)Cortical ischemia will result in reduction of, or loss of, I-wave recruitment with consequent significant attenuation or loss of MEPs on the contralateral side.
(2)Partial or complete occlusion of the leticulostriates or the anterior choroidal artery can result in rapid attenuation or loss of MEPs on the contralateral side.
The MEP is a compound muscle action potential (CMAP) recorded from the motor unit depolarized by the descending D- and I-waves (Figure 16.2). All anesthetics decrease the resting membrane potential of the αMNs; the increase in membrane potential from the combined effects of the D- and I-waves must exceed firing threshold for the αMN to depolarize. The neuromodulatory influences of serotonin and noradrenaline have a significant effect on αMN excitability by regulating channel open-state time and resting membrane potential.12 Volatile agents reduce seratoninergic output, while ketamine may potentiate it. Dexmedetomidine reduces central noradrenaline and can inhibit TcMEPs in a dose-dependent fashion.13 All of the adverse effects of anesthetic agents on MEP generation are more prominent in young children due to immaturity of the motor system. Although direct CST to αMN connections are present in the αMNs innervating muscles of the hand at birth,14 the same is not true for connections between the CST and the αMNs innervating lower extremity muscles. As a consequence of these factors, special techniques such as temporal or spatial facilitation may be required to elicit MEPs in very young children. The temporal facilitation technique, also called double-train stimulation, can be very effective for obtaining TcMEPs from young children.15, 16 Spatial facilitation is technically more difficult and is limited to the homonymous muscle in a single (or two, upper or lower) limb(s), whereas temporal facilitation has no such limits.
Figure 16.2. Transcranial MEPS. These recordings were obtained from a 2-year-old with congenital scoliosis using a double-train technique.
During complex intracranial procedures there may be selective loss of the I-waves for various reasons. Loss of the I-waves due to cortical ischemia or ischemia within the internal capsule may result in complete loss of the TcMEPs or significant attenuation of TcMEP amplitude. Compromise of blood flow to the anterior choroidal artery or the lenticulostriates can produce ischemia in the internal capsule resulting in loss or severe attenuation of the TcMEPs. Thus the TcMEPs are highly informative during procedures during which retraction near these vessels is likely to be performed. In addition to somatic muscle TcMEPs, it is possible to record TcMEPs from muscles innervated by cranial nerves, especially from CN VII,17 X,18 and XII. Special techniques are required to generate these TcMEPs, but they can be very useful during posterior fossa tumor resection involving the floor of the fourth ventricle when used in conjunction with direct motor mapping.
In some cases, the motor cortex may be directly exposed making the use of TcMEPs on the operative side impossible. In this case, either a ball probe or a grid electrode may be used to directly stimulate the motor cortex. The central sulcus can be positively identified using a phase reversal of the N20 of a cortically recorded median nerve SSEP (see above). Unlike TcMEPs, dcMEPs can be performed frequently during a procedure because there is no movement associated with the application of the stimulus.19
If tumor resections involve dissection in subcortical regions near the internal capsule, or any part of the intracranial CST, the distance between the resection margin and the CST may be accurately estimated using subcortical mapping. A ball probe or other device, such as a modified suction, can be used to deliver pulse trains of increasing current until an MEP is elicited. The current, in mA, required to produce an MEP closely approximates the distance, in millimeters, between the stimulus and the CST.20 When used in combination with dcMEPs, this technique can provide meaningful real-time feedback to the surgeon concerning the functional state of the CST and the distance between the CST and the resection margin.
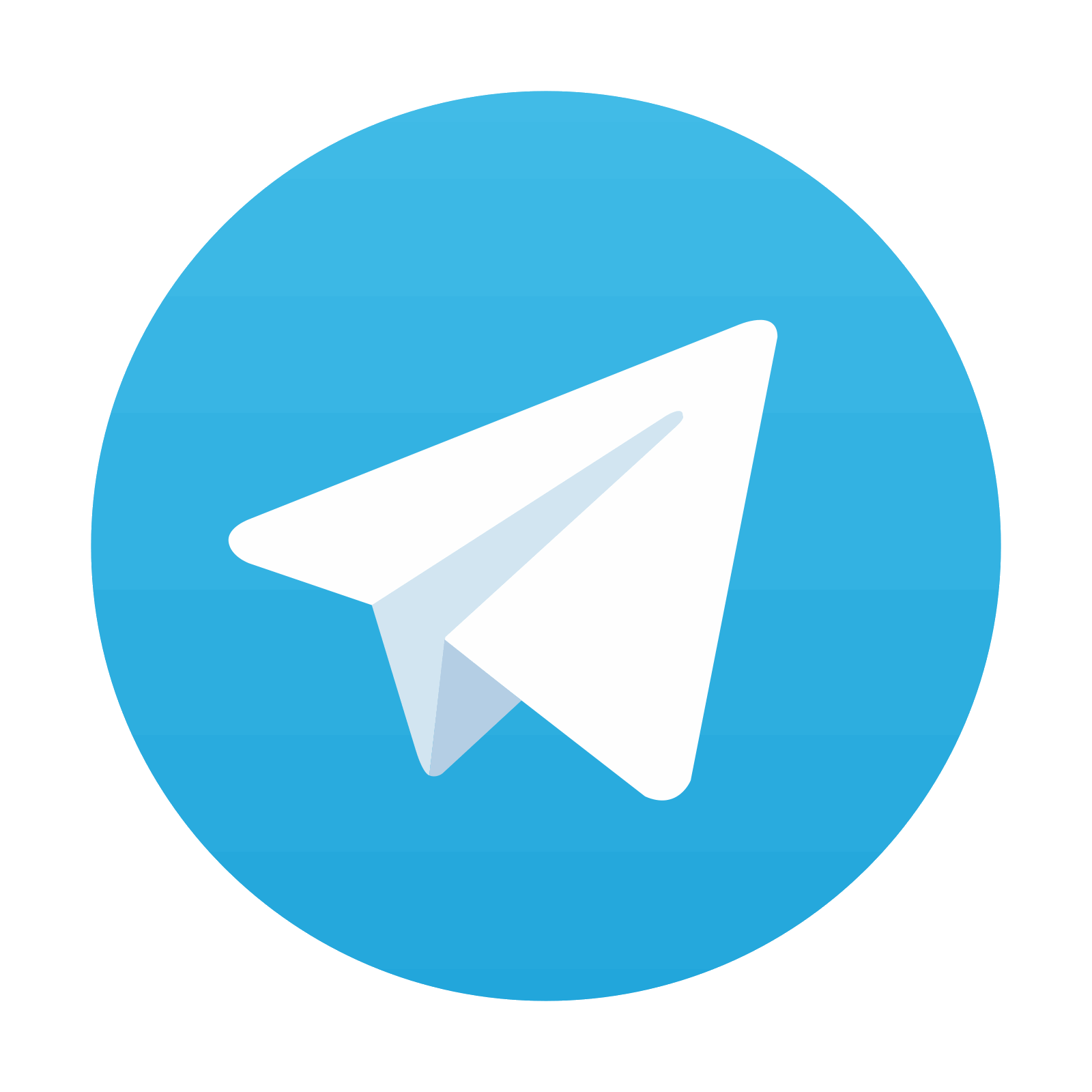
Stay updated, free articles. Join our Telegram channel
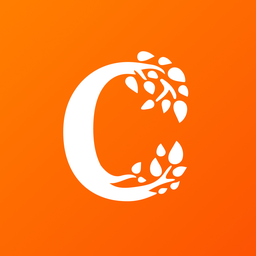
Full access? Get Clinical Tree
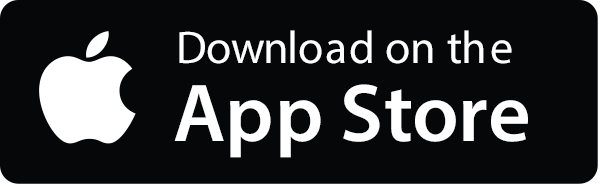
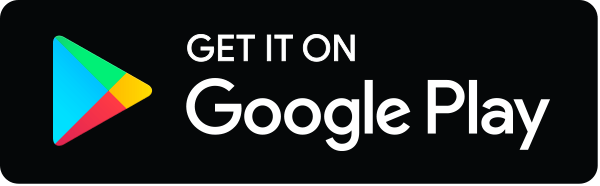
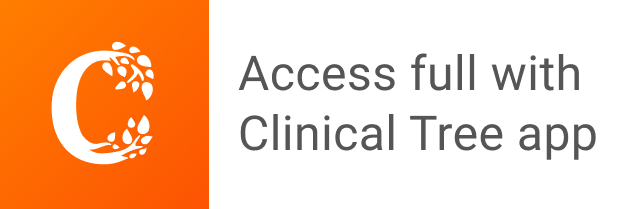