Chapter 1 Introduction
1.1 PBM: A Concept to Improve Patient Safety and Outcome
1.1.1 The Triad of Anemia, Blood Loss, and Transfusion: Three Independent Risk Factors for an Adverse Outcome
▶ Anemia. Anemia, characterized by a subnormal concentration of circulating red blood cells (RBCs), is prevalent in many different surgical populations and in critically ill patients. In patients undergoing elective surgery, the frequency of preoperative anemia ranges from 5 % to 75 %, with a higher incidence in older patients (Kulier and Gombotz 2007, Myers et al 2007, Gombotz et al 2011, Kulier et al 2014, Musallam et al 1996, Baron et al 2007). Anemia is a strong and independent predictor of adverse outcomes such as average hospital length of stay and the composite outcome of morbidity and mortality (Carson et al 2011, Nemergut et al 2004, Musallam et al 1996). One of the main contributing factors to this clinically significant condition is an absolute iron deficiency (Guralnik et al 2005). Various other factors including functional iron deficiency, chemotherapy, radiation, certain medications, menorrhagia, and congenital disorders can also cause anemia.
▶ Perioperative blood loss. Perioperative blood loss is an independent predictor for the same adverse outcomes as those seen with anemia (Carson et al 2013, Rao et al 2013, Walsh et al 2008b). Blood loss can be attributable to surgery, diagnostic or therapeutic interventions, trauma, obstetric complications, long-term anticoagulant therapy, and other reasons (Gombotz and Knotzer 2009). In nonanemic patients, acute blood loss or severe hemorrhage can lead to immediate, serious sequelae (Karkouti et al 2013, Karkouti et al 2014, Walsh et al 2008b, Hogervorst et al 2013a). In patients with preexisting anemia, the tolerance to blood loss may be even lower.
▶ Transfusion. For decades, transfusion has been considered to be the optimal treatment for anemia and/or blood loss. However, although the administration of RBCs can be lifesaving in hemodynamically unstable patients, there is little evidence of clinical benefit in hemodynamically stable patients, the group that receives the vast majority of all RBC transfusions (Bernard et al 2009, Refaai and Blumberg 2010). Although transfusions result in corrected laboratory values, they do not treat the actual cause of anemia, nor do they stop any bleeding.
Moreover, stored blood that is transfused has limited oxygen off-loading capacity (Napolitano et al 2014). Animal models have shown that the morphological changes of RBCs during storage lead to impaired tissue perfusion followed by diminished oxygenation and inadequate removal of carbon dioxide (Tsai et al 2007, Yalcin et al 2008). These rheological changes might explain the observation of a dose–response relationship between ischemic events and RBC transfusions (Murphy et al 2015, Koch et al 2006, Lacroix et al 2012).
In addition, there is a link between transfusion and cancer recurrence. The causality is still under debate, but the findings of several clinical studies are suggestive of a causal relationship (Amato and Pescatori 2013, Al-Refaie et al 2014, Riedl et al 2014, Boehm et al 2014, Luan et al 2013b, Wang et al 2007).
The relationship between transfusion-related immunomodulation (TRIM) and postoperative infection has also been the subject of scientific controversy for decades. However, in this case, the causal link has now been confirmed (Refaai and Blumberg 2009). Retrospective and prospective observational studies in various patient populations have shown a dose–response relationship between transfusion and nosocomial infections (Lacroix et al 2013, Bernard et al 2009, Carson et al 2014, Curley et al 2011). Moreover, a meta-analysis of randomized controlled trials (RCTs) comparing the use of liberal versus restrictive transfusion thresholds showed an increase in hospital-acquired infections with liberal transfusions (Rohde et al 2006).
In preparation of the 1st International Consensus Conference on Transfusion and Outcome (ICCTO), the ICCTO research group applied the Bradford Hill criteria for establishing causation to assess the link between transfusion and adverse outcomes, using the evidence available at the time (Isbister et al 2012b). The Bradford Hill criteria include strength, consistency, specificity, temporality, biological gradient or dose–response relationship (i.e., increased exposure results in increased risk), and biological plausibility of the findings. Sir Austin Bradford Hill and Sir Richard Doll used this methodology to establish the causal link between tobacco smoking and lung cancer (Darby et al 2004a). When applying the Bradford Hill criteria to transfusion and adverse outcomes, the ICCTO research group found strong evidence for a causal link. Meta-analyses of RCTs and systematic literature reviews have corroborated this finding, showing that the use of liberal—as opposed to restrictive—transfusion thresholds leads to significantly higher morbidity and mortality (Carson et al 2007) (see Chapter 1.3).
Although perceived as beneficial by many clinicians, transfusion is in fact an independent, dose-dependent, and additive risk factor for morbidity, increased hospital length of stay, and mortality in most clinical settings (Spiess 2009, Murphy et al 2015, Bernard et al 2009, Ferraris et al 2014, Howard-Quijano et al 2015, Carson 2015, Isil et al 2013). Patients at the limits of their cardiovascular reserve, such as those undergoing cardiac surgery, may constitute an exception—they have been shown to benefit from the higher hemoglobin levels achieved with a liberal transfusion threshold (Murphy et al 2013b). Overall, however, anemia, blood loss, and transfusion constitute a triad of independent risk factors for adverse outcomes (Ranucci et al 2013b, Farmer et al 1986) ( Fig. 1.1 ).

Note
Anemia, blood loss, and transfusion constitute a triad of independent predictors of an adverse outcome.
This triad constitutes a vicious circle: blood loss and bleeding induce anemia or exacerbate preexisting anemia; anemia triggers transfusion; and transfusion—as shown in several studies—increases the risk of rebleeding, potentially leading to further blood loss (Blair et al 2010, Henriksson and Svensson 2010, Hearnshaw et al 2013, Jairath et al 2013b, Restellini et al 2013, Villanueva et al 2007). The intention of breaking this cycle by modifying the potential risk factors has led to the concept of PBM.
1.1.2 PBM: Improving Outcomes by Preempting the Impact of the Triad
Rationale behind PBM
The rationale behind PBM is that improved patient safety and optimal clinical outcomes can be achieved when optimization and preservation of the patient’s own blood take priority over the transfusion of donor blood.
Note
To achieve an optimal clinical outcome, optimization and preservation of the patient’s own blood takes priority over the transfusion of donor blood.
This can be achieved by preempting the detrimental mechanisms within the triad: each of the three risk factors is modifiable and should be addressed as early as possible in the course of treatment. The clinical measures addressing these risks are grouped into the so-called three pillars of PBM:
Optimization of the patient’s endogenous RBC mass.
Proactive and timely bleeding management (minimization of diagnostic, interventional, and surgical blood loss).
Optimization of the patient’s tolerance to anemia by maximizing oxygen delivery while reducing the metabolic rate, and harnessing the patient’s ability to tolerate anemia by strictly adhering to physiological transfusion thresholds.
Note
PBM is based on three pillars. Together, they address and minimize each risk factor for an adverse patient outcome in the triad:
Optimization of the endogenous RBC mass through the targeted stimulation of erythropoiesis and the treatment of modifiable underlying disorders.
Minimization of diagnostic, interventional, and surgical blood loss to preserve the patient’s RBC mass.
Optimization of the patient-specific tolerance to anemia through strict adherence to physiological transfusion thresholds.
In most clinical scenarios, implementation of the first two pillars is sufficient to address all three risks in the triad. Through optimizing a patient’s RBC mass and reducing blood loss, the hemoglobin value of most patients is kept above the threshold where transfusion might be considered. However, addition of the third pillar can further reduce the transfusion rate (Meier and Gombotz 2013b).
1.1.3 PBM in the Surgical and Nonsurgical Setting
The concept of PBM was initially developed in the surgical setting. By applying the three-pillar approach to the pre-, intra-, and postoperative phases, the model further evolved to the multidisciplinary, multimodal nine-field matrix shown in Fig. 1.2 . Each of the fields includes a set of clinical measures that can be combined, and tailored to specific patient needs and procedures and to the expertise of a given organization.

Most PBM scenarios can be managed by a team of PBM-trained anesthesiologists, surgeons, and—in some cases—intensive care specialists. A smaller number of scenarios also require support from hematologists, gastroenterologists, radiologists, or other specialists.
Note
Each field of the multidisciplinary, multimodal nine-field matrix of PBM includes a set of clinical measures that can be combined and tailored to specific patient needs and procedures.
The principles of PBM can also be applied to procedures that have traditionally been associated with major blood loss and hemorrhage, and to medical settings where transfusion has been intrinsic to treatment. Furthermore, PBM is not confined to the transfusion of RBCs. Its principles can be expanded to preempt the transfusion of platelets, fresh frozen plasma (FFP), and other blood products that also carry risk.
Note
PBM was first developed in elective surgery but the principles can be applied in a modified form to obstetric, pediatric, and emergency surgery, trauma management, and other medical settings.
1.1.4 Brief History of PBM
The renowned heart surgeon and founder of the Texas Heart Institute, Denton Cooley, was one of the first people to develop an individualized multimodal approach to blood conservation to perform complex heart surgery without the use of RBC transfusion. The positive patient outcomes associated with this approach led to the development of comprehensive hospital-wide “blood conservation programs.” One of the early programs was established at Kaleeya Hospital in Fremantle, Western Australia, in 1990. The success of this program ultimately led to the implementation of a state-wide PBM program in Western Australia (Farmer et al 1986).
In 1994, the anesthesiologist Aryeh Shander and the clinical nurse Sherri Ozawa established a pilot program at Englewood Hospital and Medical Center in Englewood, NJ. They have reported extensively on the improved patient outcomes resulting from their programmatic approach to PBM, and their approach has become a model for numerous programs and initiatives around the world (Shander et al 2007).
The term Patient Blood Management was coined by the Australian hematologist James Isbister during a board meeting of the Medical Society for Blood Management in Prague (Farmer and Leahy 2008). He and his colleagues advocated the use of this terminology to ensure a focus on management of the patient’s own blood as opposed to the management of donor blood. In 2007, the term first appeared in the literature (Isbister 2008). In 2008, Isbister—together with colleagues from Europe—published the article “Patient blood management: the pragmatic solution for the problems with blood transfusions” (Spahn et al 2009); this was the first publication with PBM in the title. Since then, the number of PBM-related publications has been growing constantly, the articles spanning many different disciplines (Spahn et al 2009, Thomson et al 2011, Spahn 2011, Emmert et al 2011a, Gombotz 2012, Ranucci et al 2012, Gombotz 2012, Goodnough and Shander 2012, Kotze et al 2012a, Meier et al 2012b, Shander et al 2012, Shander et al 2013, Spahn et al 2013b, Bruhn 2013, Farmer et al 1986, Gombotz and Knotzer 2009, Goodnough et al 2013, Gross et al 2013, Liumbruno et al 2013, Mukhtar et al 2013, Shander et al 2013b, Shaz et al 2014, Spahn et al 2014, Cohn et al 2002, Leahy et al 2007).
The pre-, intra-, and postoperative phases of blood conservation were first described by Martyn and colleagues (2011), and the three-pillar concept of blood conservation was first mentioned in a peer-reviewed paper by Gombotz and colleagues in 2011, followed by a number of publications where this concept was referred to as the three pillars of PBM (Hofmann et al 2012). In 2008, Farmer and Hofmann combined the three phases of surgery and the three pillars of PBM to create the multidisciplinary, multimodal nine-field matrix of PBM for a PBM Program run by the Department of Health in Western Australia (Hofmann et al 2012, Hofmann et al 2013b, Farmer et al 1986). They also developed the concept of the triad of risk factors based on a review of the literature in which they identified three clinical risk factors that can be modified by PBM (Farmer et al 1986).
The Department of Health in Western Australia was the world’s first health authority to implement PBM across the public health system of an entire state or country. Other Australian health authorities followed with similar projects, and currently PBM is being implemented nationwide with the support of leading clinicians, hospital managers, and health authorities (Spahn et al 2009, Hofmann et al 2013b, Spahn et al 2013b, Farmer et al 1986).
Managed by the National Blood Authority and endorsed by the Australian National Health and Patient Research Council, five of six modules of the national Patient Blood Management Guidelines for Australia have now been developed and published online (www.blood.gov.au/pbm-guidelines). In these guidelines, the PBM Program in Western Australia is acknowledged as a pilot program that addresses many of the challenges of program implementation.
In 2010, the World Health Assembly of the WHO adopted the concept of PBM with Resolution WHA63.12: “…PBM means that before surgery every reasonable measure should be taken to optimize the patient’s own blood volume, to minimize the patient’s blood loss and to harness and optimize the patient-specific physiological tolerance to anemia following the WHO’s guide for optimal clinical use (three pillars of PBM)” (WHO 2012).
In 2014, the European Commission announced a pilot program for the implementation of PBM in five European teaching hospitals (www.europe-pbm.eu). A number of institutions in North America, Europe, and the Asian-Pacific Region are currently establishing PBM as the standard of care. An increasing number of transfusion medicine specialists and leading professional organizations—e.g., the American Association of Blood Banks (www.aabb.org/pbm)—are supporting the concept.
1.1.5 Definition of PBM
Several descriptions and definitions of PBM have been published:
“PBM is the timely application of evidence-based medical and surgical concepts aimed at achieving better patient outcome by relying on a patient’s own blood rather than on donor blood” (Hofmann et al 2012).
“Evidence-based PBM is aimed at achieving better patient outcome by relying on a patient’s own blood rather than on donor blood” (Hofmann et al 2013b).
“PBM…employs a patient-specific perioperative/peri-event, multidisciplinary, multimodal team approach to managing the patient’s own blood and haemopoietic system. PBM views a patient’s own blood as a valuable and unique natural resource that should be conserved and managed appropriately” (DHWA 2011a).
“PBM improves patient outcome by improving the patient’s medical and surgical management in ways that boost and conserve the patient’s own blood” (http://blood.gov.au/patient-blood-management-pbm).
“Patient blood management aims to improve clinical outcome by avoiding unnecessary exposure to blood components. It includes the three pillars… These principles apply in the management of any hematologic disorder. Patient blood management optimizes the use of donor blood and reduces transfusion-associated risk” (NBA 2007).
“Professional definition: PBM is the timely application of evidence-based medical and surgical concepts designed to maintain hemoglobin concentration, optimize hemostasis and minimize blood loss in an effort to improve patient outcome” (www.sabm.org).
“Public description: PBM is the scientific use of safe and effective medical and surgical techniques designed to prevent anemia and decrease bleeding in an effort to improve patient outcome” (www.sabm.org).
The various descriptions and definitions of PBM convey the idea that PBM
Is evidence-based.
Is a multimodal concept.
Is an individualized, patient-specific therapeutic approach.
Is multidisciplinary.
Is applicable in surgical and nonsurgical settings.
Improves patient outcomes.
Improves patient safety/reduces transfusion-associated risks.
Is based on three pillars.
Involves the management of the patient’s own blood as opposed to management of the patient with somebody else’s (donor) blood.
In an attempt to integrate all of these aspects, the following universal definition is proposed: PBM is an evidence-based, integrated, multidisciplinary, multimodal approach to individually manage and preserve a patient’s own blood in surgical and nonsurgical settings by correcting anemia, reducing blood loss, and harnessing and optimizing the physiological tolerance to anemia, thus minimizing or avoiding transfusion and improving patient safety and outcomes.
1.1.6 The Potential Impact of PBM
In 2011, the total number of blood units collected worldwide was approximately 92 million (WHO 2010); the number of whole blood units and RBCs transfused was probably 10 % lower (approximately 83 million). On the backdrop of this massive consumption, PBM has huge potential given the high prevalence of untreated preoperative anemia and the unmet need for bleeding management protocols—apart from protocols relating to massive bleeding (Spahn et al 2013)—and their system-wide implementation, together with a behavior-based transfusion practice that is still too liberal. Year after year, the implementation of PBM could improve the outcomes of millions of patients and help to avoid millions of allogeneic transfusions with all the associated adverse effects.
The cost of RBC transfusions to U.S. health care providers is estimated at $14 billion per annum; however, the true costs might be much higher because of transfusion-related adverse outcomes (Shander et al 2007, Hofmann et al 2015, Vamvakas 2010). A retrospective cohort study using data from the 2004 Nationwide Inpatient Sample database found that, after adjustment for confounders, the average hospital costs per patient were $17,194 higher for those who had received a transfusion than for those who had not. In terms of 2014 dollars, this would be more than $57 billion for a study population of 2.33 million inpatients who received a transfusion. A retrospective cohort study conducted in Western Australia showed that, after adjustment for potential confounders, the total hospital-associated cost of RBC transfusion reached $72 million; the study included 89,996 multiday acute-care inpatients, 4,805 of whom had received a transfusion (Trentino et al 2007).
These amounts are of macroeconomic relevance and a significant share of these costs could be reallocated, resulting in better cost-effectiveness ratios when applying PBM (Shander et al 2007).
The Austrian Benchmark Study (Gombotz et al 2011) was the first study to indicate the enormous value of PBM in elective surgery. This study showed that 97.6 %, 97.4 %, and 96.9 % of all RBC transfusions in patients undergoing total hip arthroplasty, knee arthroplasty, or coronary artery bypass (CABG) surgery, respectively, can be predicted by the following three parameters, which can all be modified through PBM (Gombotz et al 2011):
Preoperative hemoglobin value.
Blood loss.
Transfusion trigger.
These results, and the fact that most transfusions are given to hemodynamically stable patients, indicate that with PBM fully implemented, RBC transfusion will become obsolete in many clinical scenarios or it will only have a minor role. PBM programs in different parts of the world have already demonstrated significant reductions in the number of transfusions with comparable or improved patient outcomes (Moskowitz et al 2014b, Kotze et al 2012a, Frank et al 1999b).
A number of other factors are also in favor of PBM. One is the constant threat posed by emerging and re-emerging blood pathogens, even though the problems with HIV, hepatitis B virus (HBV), and hepatitis C virus (HCV) seem to have been eliminated, albeit at an enormous cost (Goodnough et al 2011, Goodnough et al 1994). Besides, a shortage of allogeneic blood in high-income countries has been predicted because of a decline in blood donations, against a background of an aging population (Greinacher et al 2007). The failure to comply with existing guidelines and the broad variability in blood consumption are a drain on health budgets, in addition to presenting an increased health risk (Sanguis Study Group 2010, Gombotz et al 2011, Bennett-Guerrero et al 2007, Gombotz et al 2012). Process cost analysis has revealed that treatment with blood products is one of the most expensive forms of modern-day therapy (Shander et al 2012, Abraham and Sun Chapter 2.3, Gombotz 2012).
Note
The high prevalence of untreated preoperative anemia and the unmet need for bleeding management protocols and their implementation, together with a transfusion practice that is too liberal, indicate that PBM represents a huge opportunity to improve patient outcomes and avoid millions of transfusions every year. The PBM approach is further supported by the inherent risks posed by new and re-emerging bloodborne pathogens, by blood supply pressures, and by the escalating costs associated with transfusion.
1.1.7 Clinical Practice: First Steps toward Reducing the Patient-specific Transfusion Risk
When implementing a PBM program, the identification and management of patients with a high transfusion probability can quickly yield initial results. Three principal steps can effectively achieve this aim:
Identification of the procedures with the highest transfusion rates/indices (see Chapter 1.6). For hospitals with a patient-level database on transfusions and blood ordering processes, it should be relatively easy to capture this kind of information.
Calculation of the mean blood loss experienced during these procedures from the hospital’s retrospective patient-level database with a representative number of cases (see 2009). Note: the calculated perioperative blood loss takes account of the amount of blood in swabs, hematomas, etc., and is therefore more accurate than blood loss estimates.
Calculation of the individual patient’s preoperative blood volume, based on the preoperative hemoglobin level, body weight, height, and gender. After deducting the hospital’s empirically collected, procedure-specific mean blood loss from the patient’s preoperative blood volume, the postoperative hemoglobin level can be predicted by using the Mercuriali algorithm. If the predicted hemoglobin level is close to, or below, the target hemoglobin level, PBM is clearly indicated. The target hemoglobin level or the transfusion threshold is set in accordance with the respective clinical picture and the transfusion guidelines of the pertinent societies (see 2009).
Note
By empirically devising and calculating the mean perioperative blood loss for selected procedures, and by using patient-specific data such as the preoperative hemoglobin level, body weight, height, and gender, patients can be identified in whom PBM is indicated.
For many institutions that wish to introduce PBM, the routine correction of anemia in patients undergoing a procedure that is associated with high blood loss might be the first step toward a change in practice. With some structural adjustments, this step can be implemented almost immediately. The adaptation of treatment modalities to systematically reduce blood loss and bleeding might require more time, particularly if this involves surgical training. However, in a fully developed PBM program, anemia should be corrected always and in every patient because it is listed as a disease in the International Classification of Diseases (ICD-10), and blood loss should always be minimized. The ultimate goal is an institution-wide change in the transfusion culture (Oliver et al 2013).
1.1.8 PBM versus Optimal Blood Use—Two Different Approaches
Knowledge of the manifold nature of the problems related to transfusion has led the majority of high-income countries to formulate guidelines, and introduce hemovigilance registries and recommendations, for the optimal use of blood products; an example is the EU Optimal Use of Blood program (www.optimalblooduse.eu) (Hofmann et al 2012). These programs must not be confused with PBM, which involves the proactive and routine identification of patients with anemia and/or those at risk of clinically significant blood loss in order to provide them with a management plan aimed at reducing these risks. As a result, PBM leads to a reduction in the demand for allogeneic RBC transfusions. By contrast, the concept of optimal blood use is product-centered. It is geared toward the provision of a pathogen-free blood supply and the minimization of blood component wastage, while its clinical scope is limited to the implementation of an optimal transfusion threshold ( Table 1.1 ).
Note
PBM reduces anemia and the demand for transfusion through improved patient care, whereas the concept of optimal blood use mainly attempts to secure the blood supply.
Conclusion
To achieve optimal clinical outcomes and improve patient safety, the management and preservation of the patient’s own blood has to take priority over the management of the patient with donor blood. This can be achieved by an evidence-based, multidisciplinary, multimodal, patient-specific treatment concept that is based on three pillars: (1) optimization of the (preoperative) endogenous RBC mass through the stimulation of erythropoiesis; (2) minimization of diagnostic, interventional, and operative blood loss to preserve the patient’s RBC mass; and (3) optimization of the patient’s physiological tolerance to anemia and strict adherence to physiological transfusion thresholds to further reduce the odds of RBC transfusion. When used in combination, these three pillars help to minimize the impact of the triad of independent risk factors for adverse outcomes (anemia, blood loss, and RBC transfusion).
The principles of PBM were first developed for elective surgery but can be applied in a modified form to obstetric, pediatric, and emergency surgery, trauma management, and medical settings. The consistent implementation of this three-pillar strategy improves the course of disease and patient safety, and leads to a substantial reduction in the use of allogeneic blood components compared with current transfusion practices.
1.2 Requirements of Modern Transfusion Medicine
1.2.1 Hemotherapy—from Blood Donation to Transfusion
The quality of modern hemotherapy does not depend solely on the quality of the blood components administered. Hemotherapy must be understood as a complex process ranging from blood donation through blood testing, indication assessment, blood sampling from the recipient, and compatibility testing to transportation to the storage site and the clinical user. Within this chain, failure of the weakest link poses risks to the patient ( Fig. 1.3 ) (Isbister 2014).

Blood Donation
Blood donors make an important voluntary and unremunerated contribution to society. Donor selection is based on medical assessment using a medical history form completed by the donor, medical interview, medical examination (at least blood pressure, pulse, temperature), and laboratory analysis (hemoglobin measurement) (e.g., Standards for Blood Banks and Transfusion Services 2013, Norfolk 2011a).
Note
Only donors who meet the minimum requirements set out in national guidelines will be permitted by the physician to donate blood.
Preparation of Blood Components
▶ Blood components. Blood transfusion in high-income countries is limited to the transfusion of blood components. RBCs, platelets, and plasma are separated from whole blood by centrifugation or collected directly from the donor through automated apheresis. Apheresis can also be used to obtain granulocytes or hematopoietic stem cells from peripheral blood.
▶ Further processing. Standard blood products can be processed further, depending on the indication. This includes irradiating blood preparations to prevent transfusion-associated graft-versus-host disease, washing cellular preparations to prevent incompatibility reactions (caused by residual plasma from the donor) in sensitized individuals, and producing separated or concentrated blood components, e.g., for use in neonatology.
Note
The use of the different blood components should be based on an individualized risk–benefit analysis, corroborated by evidence from clinical trials. Specific recommendations are given in guidelines such as the United Kingdom’s Handbook of Transfusion Medicine (Norfolk 2011a) and the Cross-Sectional Guidelines for Therapy with Blood Components and Plasma Derivatives of the German Medical Association (BAEK 2011).
Red Blood Cell Concentrates
▶ Indication. The administration of RBC concentrates continues to be a causal therapy—with the fastest onset of efficacy—for life-threatening anemic hypoxia. The indication for transfusion must be based on strict criteria adapted to the individual patient.
Note
The clinical symptoms of anemic hypoxia determine the actual indication of RBC transfusion (physiological transfusion triggers).
However, the symptoms of anemic hypoxia are nonspecific and may be difficult to identify under clinical conditions. There are no absolute or generally valid critical transfusion thresholds that can be defined for the hemoglobin level or the hematocrit. Any decision-making regarding transfusion must take into consideration not only the laboratory values, but also the duration, severity, dynamics, and cause of anemia as well as the patient’s previous history, age, and clinical status. More detailed information on the indications for, and administration of, RBC concentrates is provided in the 2011 edition of Cross-Sectional Guidelines for Therapy with Blood Components and Plasma Derivatives of the German Medical Association (BAEK 2011).
▶ Storage. RBC concentrates are stored at +4°C ±2°C for 28–49 days depending on the production process and in accordance with the manufacturer’s instructions. In recent years, there has been an extensive discussion about the extent to which the complex morphological and biochemical changes occurring during storage can affect the clinical efficacy and side effects of transfused RBC concentrates (Roback 2011b). Despite a plethora of published studies, it has not yet been possible to provide definitive answers, for two reasons: first, the studies carried out were retrospective or prospective observational studies, and second, not all influencing factors were taken into account (van de Watering 2012, van de Watering 2015). The first RCT in this area, which addressed mortality as the primary outcome, failed to show an improved clinical outcome in premature infants transfused with fresh RBCs compared with standard blood products (Fergusson et al 2014). In a 2015 study, the transfusion of fresh RBCs, as compared with standard-issue RBCs, did not decrease the 90-day mortality among critically ill adults (Lacroix et al 2012). Several other large RCTs assessing the effect of RBC storage time on mortality or multiple organ dysfunction are currently in progress and may help to shed more light on the influence of storage time (Flegel et al 2010).
Note
The question as to whether there is a causal link between the shelf life of RBC concentrates and the occurrence of adverse reactions can ultimately only be resolved by prospective randomized clinical trials.
Platelet Concentrates
Platelet concentrates contain more than 2 × 1011 platelets. Their volume is between 200 mL and 350 mL. The platelets are resuspended in either plasma or an additive solution. They are stored at +22°C ±2°C under continuous agitation.
▶ Product types. Platelet concentrates are isolated either from whole blood donations through pooling of four buffy coats from donors with the same AB0 and Rhesus D group, or through platelet apheresis from a single donor (Schrezenmeier and Seifried 2011a).
Note
In nonrefractory recipients, pooled platelet concentrates and apheresis platelet concentrates are comparable in terms of safety, tolerability, and efficacy ( Table 1.2 ) (Schrezenmeier and Seifried 2011a, BAEK 2011).
For supply reasons, both product types should be available. That way, products from donors compatible for human leukocyte antigen (HLA) and/or human platelet antigen (HPA) can be promptly administered to immunized recipients, while keeping available a supply of platelet concentrates with special characteristics.
▶ Indication. Thresholds for the therapeutic administration of platelet concentrates and for their prophylactic administration before procedures and interventions are provided in the 2011 edition of the Cross-Sectional Guidelines of the German Medical Association (BAEK 2011).
Therapeutic Plasma
Various types of therapeutic plasma are available for clinical use. The efficacy of the various product types—FFP, methylene-blue-/light-treated plasma, lyophilized human plasma, and pooled solvent/detergent-treated plasma—is broadly similar. Plasma is transfused in an AB0-compatible fashion (BAEK 2011).
▶ Indication. Plasma is used for the replacement of deficient coagulation factors (provided the missing factors are not available as factor concentrates) and for plasma exchange therapy. The specific indications have been narrowed in recent years (Shepard and Bukowski 1991, Bell et al 2011a, Rock et al 2013) and are available in the 2011 edition of the Cross-Sectional Guidelines of the German Medical Association (BAEK 2011) (see also Chapter 1.5).
1.2.2 Quality Assurance in Clinical Hemotherapy
Blood Donation Centers
Institutions that collect blood and blood components, produce blood products, and/or store and supply them, are legally required to have an effective quality assurance system in place that meets stringent safety and utility requirements.
Health Care Centers
In many countries, inpatient and outpatient centers that administer blood products are also required to set up a quality assurance system. Quality assurance comprises the entire spectrum of staffing, organizational, technical, and normative measures designed to maximize the quality of patient care and to improve it in line with the state of the art in medical science. In hemotherapy, quality indicators for the analysis and use of blood products must be defined. In addition, the qualifications and duties of the responsible persons must be defined. The quality assurance measures and operating procedures must be summarized in a quality management manual.
1.2.3 Safety of Blood Products—Adverse Reactions
▶ Classification. Based on their pathophysiological mechanisms, any adverse reactions associated with the use of blood products can generally be classified as immune-mediated or nonimmune-mediated transfusion reactions or as transfusion-related infections ( Table 1.3 ). Another classification system is based on the time course; it distinguishes between acute side effects, e.g., immediate-type hemolytic reactions, and delayed side effects, e.g., delayed-type hemolytic reactions ( Table 1.3 ). The International Haemovigilance Network provides useful definitions of adverse reactions on its website (www.ihn-org.com).
▶ Mandatory notification. Serious transfusion reactions must be reported to the manufacturer of the blood product and to the respective competent authority. Examples of reports in which the reported data are evaluated include the United Kingdom’s Serious Hazards of Transfusion (SHOT) report (Bolton-Maggs et al 2012a) and the annual hemovigilance report by the Paul Ehrlich Institute in Germany (Funk et al 2012b).
▶ Incidence. Next to acute (allergic) transfusion reactions, transfusion-related acute lung injury (TRALI) and hemolytic transfusion reaction are the most commonly reported serious transfusion-associated adverse reactions.
Note
In Germany, the risk of immunogenic TRALI has been significantly reduced since 2009 through the exclusive use of therapeutic plasma from male donors or from women with no history of pregnancy (Funk et al,2012b, Funk et al 2011, PEI 2005).
Approximately 70 % of hemolytic transfusion reactions that result in death are attributable to AB0-incompatible transfusions. Under-reporting is likely. The main reasons for mismatched transfusions are failure to identify the intended recipient, misidentification at the time of collecting the blood specimen for pretransfusion laboratory analysis, and errors when administering the transfusion, such as product mix-ups (Lippi and Plebani 2013, Stainsby et al 2006, Bolton-Maggs et al 2012a, Wallis 2000).
Note
The majority of serious transfusion reactions are caused by errors. Therefore, it is imperative to establish and verify identity at each step of the transfusion chain!
Infections
Different pathogens (viruses, bacteria, or parasites) can be transmitted via blood products.
Transfusion-transmitted Viral Infections
▶ Serology tests. Typical transfusion-transmitted viral infections include HBV, HCV, and HIV-1 infections. It was already known in the 1970s that blood product recipients can develop posttransfusion hepatitis (Barker and Gerety 2000). Since the middle of the 1970s, all blood products are screened using the HBV surface antigen (HBsAg) test for the direct detection of HBV. However, the diagnostic window is approximately 38–40 days.
▶ Nucleic acid tests. To complement serology screening tests (antibody/antigen tests), highly sensitive tests for the direct detection of viral genomes were introduced into the screening program in the late 1990s. Nucleic acid testing (NAT) is based on nucleic acid amplification techniques (e.g., real-time polymerase chain reaction [PCR] or transcription-mediated amplification) (Busch and Dodd 1999, Roth et al 2012). Germany was one of the first countries to implement this technology for blood donor screening, with significant improvements in the safety of blood products (Roth et al 2006). By 2009, more than 300 million NAT tests for HCV and HIV-1 and approximately 100 million NAT tests for HBV had been performed worldwide (Roth et al 2009). The tests resulted in the identification of 1,728, 680, and 244 blood donors who were infected with HBV, HCV, and HIV-1, respectively, and who would not have been identified with any other method.
Anti-HBc testing was introduced as an additional screening parameter in various countries (e.g., introduction in Germany in 2006) to detect occult HBV infections. Blood donors with an occult HBV infection are potentially infectious because the HBV concentration might be below the detection limit of existing screening methods (Schmidt et al 2012).
▶ Success of nucleic acid testing. Since blood donor screening for HCV by mini-pool NAT became mandatory in Germany in 1999, only one transfusion-related transmission of HCV has been observed. Likewise, the number of transmitted HIV-1 infections has markedly declined. However, in 2007 and 2010 there were isolated cases of false-negative PCR results. The amplification efficiency of the PCR technique had been reduced because of virus genome mutations in the primer/probe regions, leading to HIV-1 remaining undetected in donor plasma (Schmidt et al 2003). In two of five cases, this gave rise to the transmission of HIV-1 to a recipient (Chudy et al 2009). This diagnostic gap will be closed by using an HIV-1 NAT system with two target regions (dual-target approach).
▶ Cost-effectiveness of the “dual-safety” strategy. Apart from the evident successes achieved with the introduction of mini-pool NAT to blood donor screening, evaluation of health economic data has also highlighted the cost-effectiveness of the modern dual-safety strategy based on infection serology and NAT. This is reflected in increases in quality-adjusted life years gained thanks to the avoidance of transfusion-related HBV, HCV, and HIV transmission ( Fig. 1.4 ) (Jackson et al 2008, Nübling et al 2001).

Note
The residual risk of transfusion-transmitted viral infection is currently estimated at 1:10.8 million for HCV, 1:4.3 million for HIV-1, and 1:360,000 for HBV, based on blood products from the German Red Cross (Hourfar et al 2008).
Transfusion-transmitted Bacterial Infections
Compared with transfusion-transmitted viral infections, bacterial contamination presents a residual infection risk that is higher by a factor of 10–100 ( Fig. 1.5 ) (Blajchman and Goldman 2007, Hourfar et al 2008, Schrezenmeier et al 2002). That said, unless lethal septicemia occurs, bacteria do not cause chronic illness like the earlier mentioned viruses and the clinical relevance is therefore not easily comparable. Platelet concentrates are at particular risk of bacterial contamination because they are stored at room temperature, thus providing good growing conditions for numerous bacterial strains.

▶ Initial specimen diversion technique and shelf life restriction. The initial specimen diversion technique was introduced worldwide at the beginning of this century to reduce the risk of bacterial transmission (de Korte et al 2011, de Korte et al 2009). This means that subsequent to venipuncture, the first 30 to 40 mL of blood are diverted into a separate bag. Bacteria introduced by the needle puncture will thus be removed again with the initial blood flow. This technique has helped to reduce the residual bacterial infection risk by around 50 %.
Studies carried out worldwide found that the prevalence of bacterial contamination of platelet concentrates is 1 in 2,000. The incidence of serious septic reactions to platelet concentrate transfusions is reported to be between 1 in 50,000 and 1 in 150,000. For Germany, the Paul Ehrlich Institute reported a total of 12 deaths because of bacterial transmission via blood components between 1997 and 2009 (with a total of approximately 50 million transfused blood products). Although platelet concentrates constitute less than 8 % of all transfused units, they were implicated in six cases, with most of these platelet concentrates having been transfused at the end of their shelf life. Consequently, the maximum shelf life for platelet concentrates in Germany was reduced from 5 days to 4 days.
▶ Detection and inactivation procedures. Worldwide, culture methods (de Korte 2011) (e.g., BacT/Alert or Bactek) or rapid test methods including PCR-based detection methods (Dreier et al 2005, Sireis et al 2009) are the main methods currently used to screen for bacterial contamination. As an alternative, pathogen inactivation methods—e.g., Mirasol (AuBuchon et al 2011), Intercept (Osselaer et al 2015), and Theraflex (Seltsam and Müller 2012)—are being developed. Methods based on a photochemical or photodynamic functional principle lead to the irreversible destruction of DNA, thus preventing pathogen growth. A drawback that persists to the present day is that different pathogen inactivation procedures are needed for different blood products.
Note
The risk of transfusion-transmitted bacterial infections was significantly reduced through introduction of the initial specimen diversion technique, the reduction of the maximum shelf life for platelet concentrates from 5 days to 4 days, and the introduction of detection techniques for bacterial contamination.
Transfusion-associated “Proteinopathies”
Transfusion-associated Variant Creutzfeldt–Jakob Disease
Creutzfeldt–Jakob disease (CJD), a disease that has been known for several decades but is very rare, belongs to the group of diseases associated with irreversible damage to the central nervous system and involves the deposition of pathological prion proteins. To date, there is no spontaneous cure or treatment. CJD remains an important health problem because of the emergence of a new variant of this disease in the United Kingdom in 1996. Variant CJD (vCJD) has been linked to foodborne transmission to humans of an infectious agent contracted through the consumption of meat products derived from cattle infected with bovine spongiform encephalopathy (BSE), also known as classical BSE, which was first reported in the United Kingdom in 1986.
▶ Epidemiology. vCJD is very rare. By February 2015, 229 people had contracted vCJD worldwide, 177 of whom in the United Kingdom, 27 in France, 5 in Spain, 4 each in Ireland and the United States, 3 in the Netherlands, 2 each in Italy, Portugal, and Canada, and 1 each in Japan, Taiwan, and Saudi Arabia (NCJDRSU 2012). The number of vCJD cases in the United Kingdom peaked in 2000 with 28 deaths. There has since been a sharp decline in the disease rate, with a drop to fewer than five new infections per year worldwide. So far, no cases of vCJD have occurred in Germany, Austria, or Switzerland, but the emergence of new cases in other countries cannot be ruled out.
▶ Bloodborne transmission. Animal experiments have demonstrated that bloodborne transmission of prions is in principle possible. Four human cases (including one “subclinical case”) where prions are thought to have been transmitted in non-leukocyte-reduced RBC concentrates have been reported in the United Kingdom. This must be viewed as proof of the potential human-to-human transmissibility of the vCJD agent through blood. The patients had received blood products from donors in whom vCJD was diagnosed 17, 18, 20, and 41 months after the implicated donation. It should be noted, however, that these patients themselves were exposed to the same risk factors for vCJD as their donors so that the connection to blood transfusion is not definitive.
▶ Measures. It can be assumed that new vCJD infections from the food chain have been effectively suppressed since 1996. One of the measures contemplated to block any ongoing hypothetical transmission chain and the persistence of vCJD via blood products was the exclusion of transfusion recipients from blood donation. This measure was introduced in some countries (the United Kingdom, the Netherlands, Switzerland, and France, although in the last case this measure had already been imposed in 1998 in the wake of cases of viral transmission). However, a model calculation based on pessimistic assumptions showed that the general exclusion of transfusion recipients would not essentially impact epidemiological trends, and any potential effect in terms of preventing isolated cases would, at most, be minimal. Since exclusion of a significant number of blood donors would have a negative effect on the blood supply, the exclusion of transfusion recipients from blood donation was not implemented in Germany.
Some countries have opted to indefinitely defer individuals from donation who spent time in the United Kingdom (or in Europe) during the critical time window. In the United Kingdom, patients born after the emergence of vCJD receive non-European plasma products.
Any secondary transmission could be suppressed as far as possible if a screening test were available. No such test is available at present, nor will it be in the foreseeable future. Therefore, top priority should be given to the development of such test methods.
Alzheimer’s Disease—a “Novel Transfusion-associated Proteinopathy”?
At the beginning of 2012, media reports caused a sensation with headlines suggesting that researchers studying Alzheimer’s disease had found evidence that this type of dementia can be transmitted through RBC transfusion. American neuroscientists were reported to have transmitted Alzheimer’s disease through blood from a mouse with a model of this condition to another healthy mouse (Morales et al 2012).
Even though the experiments in transgenic, predisposed mice did produce evidence of an accelerated induction of cerebral amyloid deposition that was potentially transfusion-mediated, it remains unclear whether this led to the development of the disease in the animal models. There is convincing evidence that Alzheimer’s disease belongs to a group of “protein misfolding diseases” subsumed under the newly coined collective term proteinopathies (de Calignon et al 2011, Soto 2013). In these diseases, misfolded proteins (in Alzheimer’s disease this is amyloid β) with an altered structure serve as a “template” for the misfolding of normally folded proteins; the misfolded proteins then form aggregates that are thought to damage brain tissue. Based on this hypothesis, misfolded amyloid β serves as a “seed” (Jucker and Walker 2012). Hence, certain parallels to prion diseases (CJD, vCJD) can be drawn ( Table 1.4 ), but these have not yet been studied in detail, let alone proven. In a recent evaluation of the potential infectivity of proteins associated with Alzheimer’sor Parkinson’s disease (i.e., amyloid β, tau, and α-synuclein) in 796 recipients of cadaveric human growth hormone, a population with a markedly elevated risk of vCJD, no cases of Alzheimer’sor Parkinson’s disease were identified (Irwin et al 2011).
Note
There is no evidence of human-to-human transmissibility of Alzheimer’s disease. The available evidence of experimental bloodborne transmission must be viewed as provisional, but should be urgently reviewed in light of the experience with vCJD. Experimental and epidemiological data are needed to that effect. To date, there is no epidemiological evidence of a link between transfusions and Alzheimer’s disease but, equally, no specific studies have been carried out to address this question.
1.2.4 Outlook
Demographic Trends
The European population is aging rapidly (WHO Regional Office for Europe 2011). The group aged 65 years or older constituted 15 % of the total population by the end of 2010 and is projected to comprise more than 25 % by 2050, so it is the fastest-growing segment of the population. A similar demographic change is expected for the United States; the population aged 65 and older increased from 8.1 % of the total population in 1950 to 12.8 % in 2009 and is projected to reach 20.2 % in 2050 (Shrestha and Heisler 2011).
Note
According to the projected demographic changes, the drop in the proportion of younger potential donors will coincide with a growing need for blood products because of more invasive treatments for the expanding group of older people ( Fig. 1.6 ) (Seifried et al 2012b).

Modern Treatment Methods
Modern methods of treatment will help to restrict the consumption of blood and blood products. Various perioperative measures are already in use, such as blood salvage from the surgical site, use of controlled hypotension, and optimized peri-operative anticoagulation management. Thanks to technological advances, it is now increasingly possible to avail of minimally invasive surgery for procedures involving a risk of bleeding. Targeted therapeutic antibodies, tyrosine kinase inhibitors, and novel targeted cancer drugs will, in some cases, replace myelosuppressive chemotherapy regimens, thus reducing the need for blood. At present, hemoglobin- or perfluorocarbon-based oxygen carriers are rarely used because of side effects and intolerance reactions, but the development of erythropoietin and thrombopoietin analogues has meant that blood products can be avoided for certain indications.
In parallel with the development of recombinant proteins, which have already replaced some plasma-derived therapeutic proteins, a similar trend can be expected for cellular blood components. Even the exvivo production of RBCs seems increasingly more realistic thanks to advances in stem cell research. However, at present the development of universally deployable RBC concentrates from stem cells appears difficult, if not impossible, given the large number of clinically relevant blood groups, the broad genetic diversity of blood group alleles, and the resulting diversity of potential alloantibodies in transfused patients. RBC production from stem cells is currently performed on a laboratory scale. There are still many problems to be resolved in this respect, including: industrial-scale production in compliance with Good Manufacturing Practice; prevention of pathogen transmission from immortalized stem cell cultures; avoidance of malignant transformation; quality control of manufactured products (e.g., absence of nucleated RBCs); and approval and monitoring criteria. To produce RBC concentrates from stem cells of Good Manufacturing Practice quality using the current production processes, several hundred liters of high-grade culture medium—and commensurate financial resources—would be needed (Seifried et al 2012b).
Note
Novel therapeutics and conventional blood products must be evaluated in comparative clinical trials so that evidence-based treatment recommendations can be further developed.
Conclusion
Life expectancy in high-income countries has continued to increase for various reasons, including high-performance medicine. Hemotherapy is one of the key elements of modern treatment concepts. Without the transfusion of RBC and platelet concentrates and of therapeutic plasma, it would not be possible to carry out high-dose chemotherapy for hematologic and solid tumors, hematopoietic stem cell transplantation, organ transplantation, major surgical procedures, or modern trauma management. Accordingly, the annual requirement for blood products has continued to rise during the last decades in many countries. The demands made on modern transfusion medicine include the assurance that hemotherapy is as safe as possible and that the clinical indications for the use of blood products are based on evidence. However, the safety of hemotherapy is not determined by blood products alone but also, to a large extent, by the quality of the transfusion process. Hemovigilance data from the United Kingdom (SHOT) and Germany (Paul Ehrlich Institute) show that there is still room for improvement, in particular as regards the indications for and the use of blood products (Funk et al 2011, Bolton-Maggs et al 2012a). Thanks to the development of modern screening methods, stringent donor selection criteria, and pathogen inactivation methods, blood products today have reached a safety level never witnessed before in the history of medicine.
1.3 Transfusion and Patient Outcomes
1.3.1 Introduction
Any review of the literature on transfusion and patient outcomes is sobering. In nonbleeding patients, evidence for the benefit from transfusion is scant—even in critically ill patients. Of concern is the large and growing body of literature showing that transfusion is independently associated with adverse patient outcomes in a dose-dependent manner ( Table 1.5 ) (Hofmann et al 2012, Isbister et al 2012b).
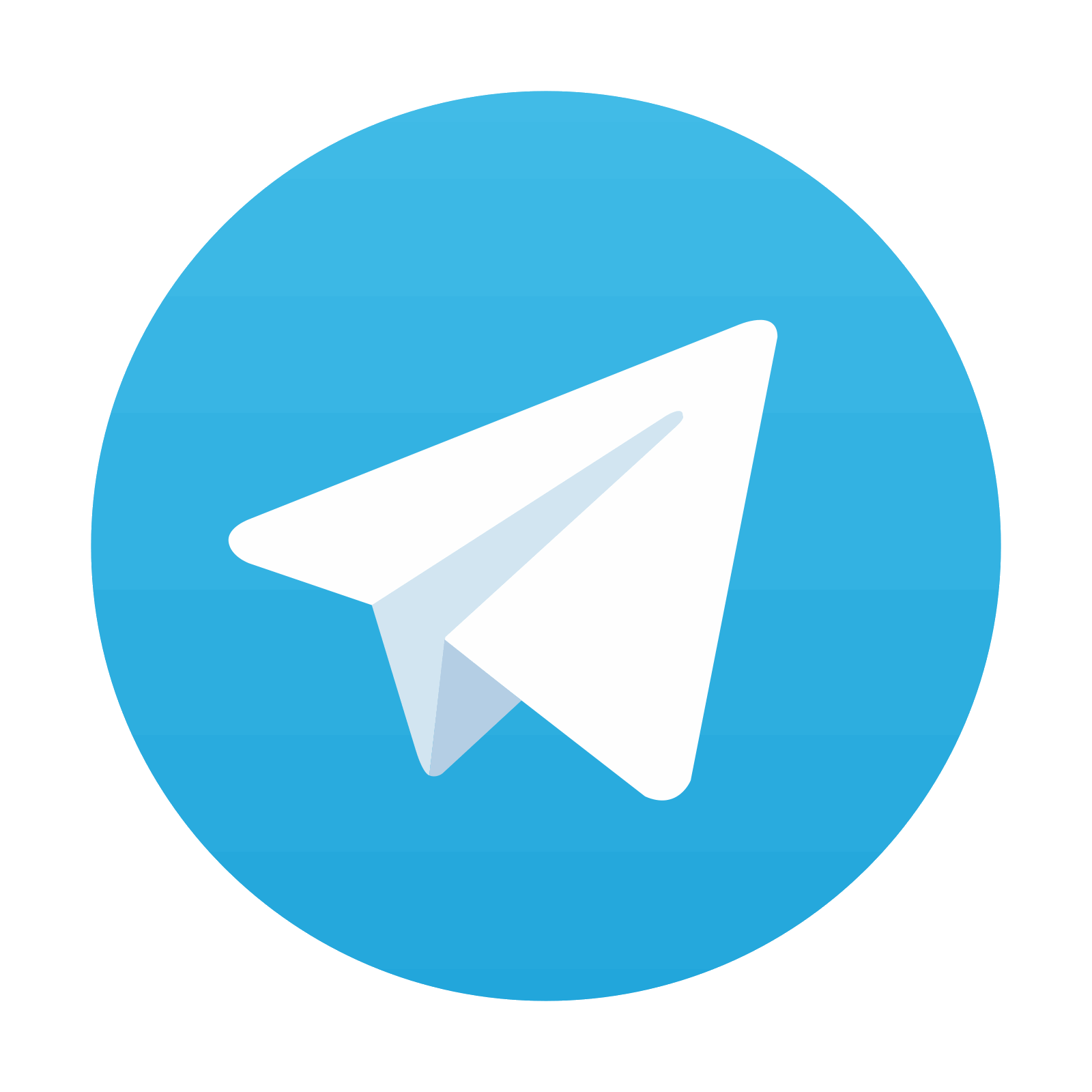
Stay updated, free articles. Join our Telegram channel
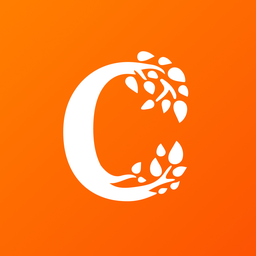
Full access? Get Clinical Tree
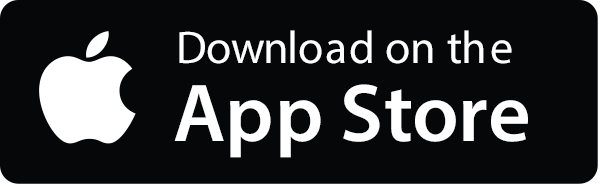
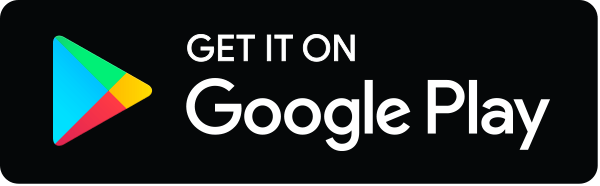
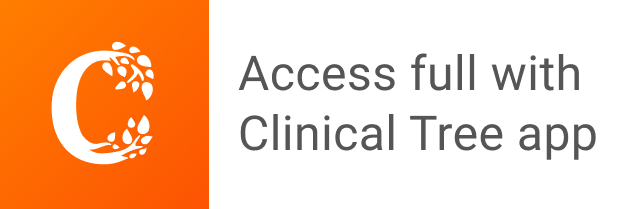