Introduction
The anesthetic management of infants and children undergoing neurosurgical procedures should be based on the developmental stage of the patient. The evolving maturational changes of the various organ systems have a significant impact of the drugs and techniques used for the safe conduct of anesthesia. Subspecialty training in pediatric neurosurgery has driven advances in intracranial surgery in infants and children, prompting calls for similarly trained anesthesiologists and intensivists for the management of these infants and children.1 Age-dependent differences in cranial bone development, cerebrovascular physiology, and neurologic lesions distinguish neonates, infants, and children from their adult counterparts. In particular, the central nervous system (CNS) undergoes a tremendous amount of structural and physiological change during the first two years of life. Neurosurgical lesions and associated surgery in this vulnerable age group have been linked to increased mortality and decreased academic achievement scores at adolescence.2 Furthermore, outcome studies reveal increased perioperative morbidity, mortality, and cognitive deficits in this patient population.3–5 This chapter highlights these age-dependent differences and their effect on the anesthetic management of the pediatric neurosurgical patient.
Developmental Aspects
Central Nervous System
The CNS develops early in gestation and is orchestrated by a combination of transcriptional and mechanical factors.6 A basic understanding of normal and abnormal development of the CNS is essential for comprehending the pathology of congenital lesions of the CNS.7
The primitive CNS is derived from the neural plate, which folds and fuses dorsally. Primary neurulation occurs when the neural plate folds to form the neural tube. The walls of the neural tube give rise to the brain and spinal cord, while the canal develops into the ventricles and central canal of the brain and spinal cord, respectively. Fusion of the cranial neural folds and closure of the cranial neuropore, which give rise to the forebrain, midbrain, and hindbrain, arise from these structures. Failure of the anterior neuropore to close by 24 days results in anencephaly. Secondary neurulation ensues when the neuroepithelium caudal to the posterior neuropore closes. Derangements in this progression can lead to spinal dysraphism (spinal bifida, myelomeningocele, and tethered cord).
After birth, the CNS is essentially fully developed, with some fine tuning that occurs between the neonatal and toddler periods.8 Cerebral blood flow (CBF) varies with the age of the patient, which peaks between 2 and 4 years and settles to adult levels at 7–8 years.9 Prematurity, traumatic brain injury, neurovascular anomalies, hypoxic brain injuries, intracranial hemorrhage, inflammatory processes, and congenital heart defects have an impact on cerebral hemodynamics. Although the theoretical autoregulatory range of blood pressure in infants is lower than in adults due to the relatively low cerebral metabolic requirements and blood pressure during infancy, recent evidence demonstrates heterogeneity of the lower limits of autoregulation in pediatric pateints.10 Although cerebral autoregulation is intact in healthy full-term neonates,8 it may be absent in critically ill premature neonates.11 CBF pressure-passivity is common in premature neonates with low gestational age and birth weight and systemic hypotension. Systolic arterial blood pressure is a poor surrogate of cerebral perfusion pressure in these patients, and the diastolic closing pressure may be a better measure of cerebral perfusion in this population.12 Extremes in blood pressure can lead to cerebral ischemia and intraventricular hemorrhage and dictate rigorous control of hemodynamics in this vulnerable population.
Children have high cerebral metabolic requirement for oxygen and glucose (CMRO2 and CMRGlu) relative to adult values (CMRO2 5.8 vs. 3.5 mL/100g/m and CMRGlu 6.8 vs. 5.5 mL/100g/m, respectively).13 At birth, CMRGlu is approximately 13–25 μmol/100g/min and rises to 49–65 μmol/100g/min at 3–4 years. It remains at this rate until 9 years and subsequently settles at 19–33 μmol/100g/m.14 These ontological changes in CRMO2 and CMRGlu are reflected in CBF values derived from brain perfusion computed tomographic (CT) scans.9 Cerebrovascular reactivity to carbon dioxide appears to be normal in newborns, but may be deranged in the setting of perinatal asphyxia.15 Inspired concentrations of oxygen (FIO2) have an impact on CBF. Decreasing FIO2 from 1.0 to 0.21 decreases CBF by 33%.16 Premature neonates are also vulnerable to the detrimental effects of high FIO2 due to liberation of reactive oxygen species leading to bronchopulmonary dysplasia and retinopathy of prematurity.17
Neonates and infants initially have compliant intracranial space due to several open fontanelles, which close in sequence from 4 months to 1 year. Therefore, gradual increases in intracranial mass due to tumor, chronic hydrocephalus, and hemorrhage are undetectable due to compensatory distension of the fontanelles and widening of the cranial sutures. However, given the diminutive neonate and infant intracranial volume, acute increases in cranial content due to blood or cerebrospinal fluid often result in life-threatening intracranial hypertension.18
Cardiac System, Including Transitional Circulation
The cardiac system is an integral component of neurovascular development, and factors that affect changes in the growth and development of the heart, including the changes during transitional circulation from intrauterine to extrauterine conditions, will also affect the brain and CNS. The neonatal myocardium is immature and incompletely developed with fewer muscle cells and more connective tissue compared to adults, which results in poor ventricular compliance and limited response to increasing preload to augment cardiac output. The high metabolic rate of neonates requires a proportional increase in cardiac output. Furthermore, the neonatal heart functions at close to its maximal rate and stroke volume just to meet basic oxygen demand. This makes the immature myocardium very susceptible to hypocalcemia, which is exacerbated by increased citrate from administration of blood products and albumin, as well as increased sensitivity to volatile anesthetics and calcium channel blockers.
Pediatric Airway
Anatomic differences between the pediatric and adult airway are primarily due to the size and orientation of the upper airway, larynx, and trachea. Neonates and infants have the greatest differences from adults in this respect, with the configuration of the larynx becoming similar to that of adults after the second year of life. An infant’s larynx is funnel shaped and narrowest at the level of the cricoid, thus making this region the smallest cross-sectional area in the infant airway. This places the infant at risk for life-threatening subglottic obstruction secondary to mucosal swelling after prolonged intubation with a tight-fitting endotracheal tube. An endotracheal tube can also migrate into a mainstem bronchus if the infant’s head is flexed for a suboccipital approach to the posterior fossa or the cervical spine. Therefore, the anesthesiologist should auscultate both lung fields to rule out inadvertent intubation of a mainstem bronchus after the patient is positioned for the surgical procedure.
Renal and Hepatic System
Renal blood flow doubles during the first 2 weeks of postnatal life, when adjusted for body surface area, and continues to increase until it reaches adult values by 2 years. The glomerular filtration rate (GFR) also doubles over the first 2 weeks of life and continues to increase until 1 to 2 years. As a result, neonates are much less able to conserve or excrete water compared to older children and adults; thus meticulous attention must be paid to fluid management during long cases and cases with significant blood loss or fluid shifts. In addition, neonates are also inefficient at excreting potassium, and the normal range of potassium may be higher in neonates and children compared to adults. Neonates in particular maintain a slightly acidotic pH (7.37) and have lower plasma bicarbonate concentrations (22 mEq/L) compared to older children and adults (pH 7.39 and bicarbonate 22 mEq/L). Thus, while neonates are able to maintain acid-base homeostasis, they are less able to buffer larger acid loads.
At birth the liver is structurally and functionally immature, with the neonatal liver containing 20% fewer hepatocytes, which are each only half the size of adult hepatocytes; the liver is even more underdeveloped in preterm infants. Due to the relative immaturity of the neonatal liver, drug metabolism and excretion as well as glucose management can be significantly affected. A dextrose-containing intravenous fluid may be used for maintenance in long cases with neonates given the immature liver’s limited ability to store and utilize glycogen.
Hepatic drug metabolic activity appears as early as 9 to 22 weeks. Drug metabolism by the liver usually involves enzymatic conversion of medications from a lipid soluble (less polar) state into a more water-soluble (more polar) state. Phase I reactions transform drugs via oxidation, reduction, or hydrolysis. The cytochrome P450 (CYP) enzyme system provides most of the phase I drug metabolism for less polar (lipophilic) compounds. Neonates have a reduced total quantity of CYP enzymes, with activity 50% of adult values. This reduction in CYP decreases clearance for many drugs, including theophylline, caffeine, diazepam, phenytoin, and phenobarbital.
Phase II reactions transform drugs via conjugation reactions such as sulfation, acetylation, and glycuronidation. Activity of uridine diphosphoglucuronosyltransferases (UGT), which is responsible for glucuronidation of bilirubin and many medications like morphine, acetaminophen, dexmedetomidine, and lorazepam, is limited immediately after birth and different isoforms mature at different rates with growth and development, but in general adult activity levels are reached by 8 to 18 months of age.
The immature liver also has a limited capacity for protein synthesis, which decreases the proportion of drugs bound to proteins in circulation and increases the amount of free drug. Acidic drugs (e.g., diazepam) mainly bind to albumin, while basic drugs (e.g., amide local anesthetic drugs) bind to globulins, lipoproteins, and glycoproteins. Due to decreased synthesis of all proteins, both basic and acidic drugs can have significantly greater effects in neonates than adults.
In addition to differences in hepatic enzymatic activity, hepatic blood flow and body composition also significantly affect enzymatic drug degradation. As neonates mature, a larger proportion of the cardiac output is delivered to the liver, thus increasing drug delivery and subsequent degradation. In general, the half-lives of medications that are cleared by the liver are increased in neonates, decreased in children 4 to 10 years of age, and reach adult levels in adolescence. Preterm and term infants have proportionally more water than older children and adults, which increases the volume of distribution of water-soluble drugs. However, neonates oftentimes are more sensitive to the neurologic, respiratory, and cardiovascular effects of many medications, and a lower dose may be needed to achieve the desired effect. Preterm infants are particularly susceptible to anesthetic medication and may require even smaller blood concentrations to achieve the desired effect.19 Due to the complexity of differences in enzymatic activity, circulatory volume, and variable neurologic and hemodynamic responses to anesthetic medications, it is important to titrate the dose of all medications to the desired response to all preterm and term infants.
Preoperative Assessment and Planning
Neonates and infants have the highest risk for perioperative respiratory and cardiovascular morbidity and mortality of any age group.20, 21 They are particularly sensitive to the depressant effects of general anesthesia and the physiological stress of surgery. Therefore, a thorough review of the patient’s history can reveal conditions that may require further evaluation and be optimized before surgery (Table 1.1). An echocardiogram and consultation by a pediatric cardiologist may be needed to optimize cardiac function prior to surgery. The neonatal respiratory system may be challenging due to the diminutive size of the airway, neonatal pulmonary physiology, craniofacial anomalies, laryngotracheal lesions, and acute (hyaline membrane disease, retained amniotic fluid) or chronic (bronchopulmonary dysplasia) disease. These conditions are in a state of flux as the patient matures.
Table 1.1 Coexisting Conditions That Impact Anesthetic Management
Condition | Anesthetic Implications |
---|---|
Congenital heart disease | Hypoxia |
Arrhythmias | |
Cardiovascular instability | |
Paradoxical air emboli | |
Prematurity | Postoperative apnea |
Gastrointestinal reflux | Aspiration pneumonia |
Upper respiratory tract infection | Laryngospasm, bronchospasm hypoxia, pneumonia |
Craniofacial abnormality | Difficult tracheal intubation |
Denervation injuries | Hyperkalemia after succinylcholine |
Resistance to non-depolarizing muscle relaxants | |
Abnormal response to nerve stimulation | |
Epilepsy | Hepatic and hematological abnormalities |
Increased metabolism of anesthetic agents | |
Ketogenic diet | |
Arteriovenous malformation | Congestive heart failure |
Neuromuscular disease | Malignant hyperthermia |
Respiratory failure | |
Sudden cardiac death | |
Chiari malformation | Apnea |
Aspiration pneumonia | |
Hypothalamic/pituitary lesions | Diabetes insipidus |
Hypothyroidism | |
Adrenal insufficiency |
Preoperative fasting guidelines have evolved and are frequently dictated by regional practices.22 The purpose of limiting oral intake is to minimize the risk of aspiration of gastric contents on induction. However, prolonged fasting periods and vomiting may induce hypovolemia and hypoglycemia, which can exacerbate hemodynamic and metabolic instability under anesthesia.
Intraoperative Management
Induction of Anesthesia
A smooth transition into the operating suite depends on the level of anxiety and the cognitive development and age of the child.23
Induction of anesthesia should be dictated by the patient’s developmental stage, comorbidities, and neurologic status. Children between the ages of 9–12 months and 6 years may have separation anxiety. Midazolam, administered orally or intravenously, is effective in relieving anxiety and producing amnesia. Parental presence during induction of anesthesia is common in pediatric operating rooms and requires full engagement of the surgical team. If the patient does not have intravenous access, anesthesia can be induced with sevoflurane, nitrous oxide, and oxygen by mask. However, intracranial hypertension may be exacerbated if the airway becomes obstructed during induction. Maintenance of a patent airway with mild hyperventilation will alleviate this problem. In patients with intravenous access, anesthesia can be induced with a propofol. It should be noted that significant hypotension and possible cerebral ischemia might occur after an induction dose of propofol,24 due to the lack of surgical stimulation or in the setting of hypovolemia. This can be minimized by reducing the dose of propofol and adjuvant opioids for induction and an intravenous fluid bolus. Some patients presenting for neurosurgical procedures may be at particular risk for aspiration of gastric contents, and a rapid-sequence induction of anesthesia with succinylcholine is required to expeditiously intubate the trachea. Contraindications to the use of succinylcholine include malignant hyperthermia susceptibility, muscular dystrophies, and recent denervation injuries.
Airway Management
Since the head and airway are inaccessible to the anesthesiologist during most neurosurgical procedures, tracheal intubation requires careful planning. Orotracheal intubation is acceptable for most neurosurgical procedures, especially for surgical approaches to the supratentorial area and when transsphenoidal exposure is planned. However, patients in the prone position are at increased risk for kinking of the orotracheal tube and macroglosia due direct pressure injury to the tongue. Nasotracheal tubes are best suited for these situations because they are easily secured and less likely to be kinked or dislodged during long procedures.
Vascular Access and Positioning
Given the diminutive size of pediatric patients and limited access, the patient should be positioned with all vascular access, monitors, and endotracheal tube secured prior to the start of surgery. Large peripheral venous cannulae are sufficient for most craniotomies. Central venous cannulation may be necessary. Femoral vein catheterization avoids the risk of pneumothorax and does not interfere with cerebral venous return. Furthermore, femoral catheters are easily accessible. The routine use of central venous catheters was not effective in reducing hypotension in craniofacial surgeries in pediatric patients.25 Given the small caliber of an infant cerebral venous catheter, its use as a conduit for aspiration of venous air embolism (VAE) is questionable.26 Placement of peripherally inserted central catheter (PICC) through the cephalic, basilica, or brachial veins and advanced to the distal superior vena cava under ultrasound guidance has the advantage of being a noninvasive and long-term access to the central circulation.27 Cannulation of the radial artery provides direct blood pressure monitoring and sampling for blood gas analysis. The dorsalis pedis and posterior tibial artery are more accessible should attempts at radial artery cannulation fail.
Patient positioning requires careful preoperative planning to allow adequate access to the patient for both the neurosurgeon and anesthesiologist. Children have relatively thin skulls and are at risk for depressed skull fractures and perforation of underlying blood vessels with cranial fixation devices.28, 29 Should a depressed skull fracture or intracranial hemorrhage be suspected, urgent imaging with CT or magnetic resonance imaging (MRI) scanning can detect the extent and location of the injury and prompt immediate evacuation of the hematoma.30 Modifications of these standard fixation devices and headrests should be utilized to minimize this preventable and potentially lethal complication.31
Surgical positioning affects the physiologic status of the patient (Table 1.2). The prone position can increase intra-abdominal pressure and lead to impaired ventilation, venocaval compression, and bleeding due to increased epidural venous pressure. Soft rolls are generally used to elevate and support the lateral chest wall and hips in order to minimize abdominal and thoracic pressure. Neurosurgical procedures performed with the head slightly elevated facilitate venous and cerebrospinal fluid (CSF) drainage from the surgical site. However, this increases the likelihood of VAE. Significant rotation of the head can also impede venous return via compression of the jugular veins and can lead to impaired cerebral perfusion, increased intracranial pressure (ICP), and venous bleeding. Vigilant assessment of the airway is critical in prone patients throughout the case because of the propensity for the tongue to slide out of the mouth and kink the endotracheal tube. Nasotracheal intubation and placement of an oral bite/tongue may prevent these complications. Obese patients may be difficult to ventilate in the prone position and may benefit from a sitting position. In addition to the physiological sequelae of the sitting position, a whole spectrum of neurovascular compression and stretch injuries can occur.
Table 1.2 Physiologic Effects of Patient Positioning
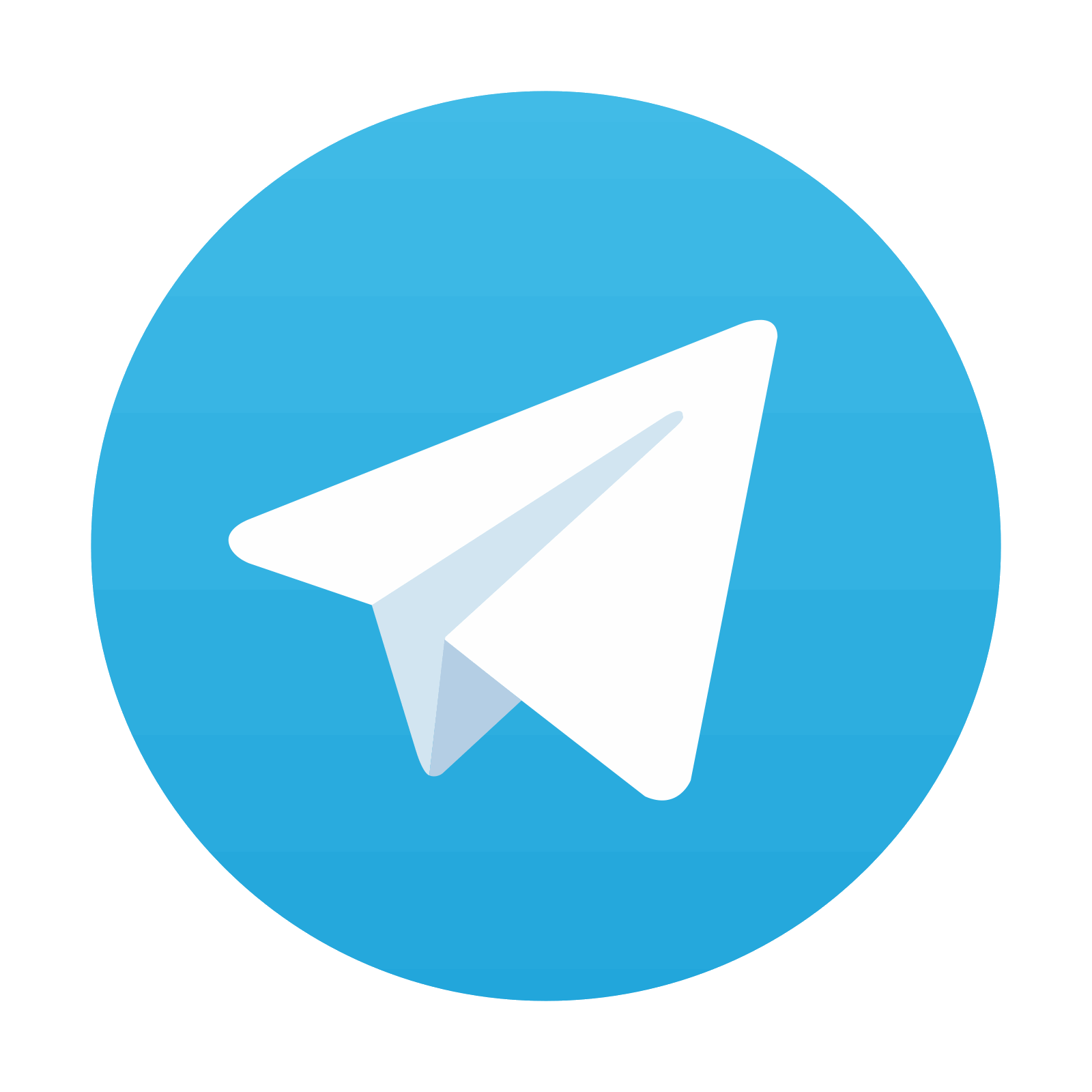
Stay updated, free articles. Join our Telegram channel
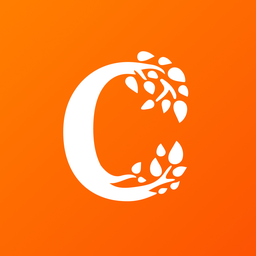
Full access? Get Clinical Tree
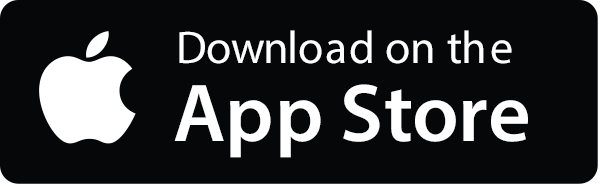
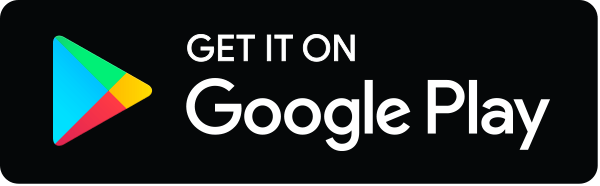
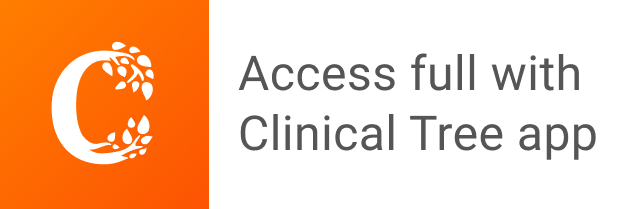