Acknowledgments
The authors would like to express their gratitude and great respect for the late William L. Young, MD, James P. Livingston Professor and Vice Chair, Department of Anesthesiology, University of California, San Francisco. Bill was an author of this chapter in the last three editions of this book. Bill’s unsurpassed clarity of thought laid the foundations for this chapter in its current form. Bill’s sage advice will always be missed but his vision and contributions to cerebral blood flow physiology will inspire future generations of clinicians and researchers.
The author would also like to thank Mei Wang, MPH, Research Associate Scientist, in the Department of Anesthesiology, College of Physicians & Surgeons of Columbia University, New York, who as with the previous edition, helped in preparation of the manuscript.
Studies of cerebral circulation have improved the understanding of the function and pathophysiology of the central nervous system (CNS). The purpose of this chapter is to review the basic mechanisms of CNS circulatory behavior and the tools used to understand them. The chapter begins with a discussion on regulation of cerebral blood flow in health and the failure of regulation in disease states, and proceeds to discuss the methodology for measuring cerebral blood flow (CBF). A discussion of spinal cord blood flow follows; the chapter ends with a discussion of the applied aspects of manipulating cerebral blood flow and monitoring CBF in the clinical setting.
Physiology of the cerebral circulation
Regional Cerebral Blood Flow Requirements
The lack of a substrate reserve in the CNS and its inability to sustain anaerobic metabolism for more than a few minutes requires a constant blood flow that is finely tuned to the metabolic needs of the tissue. The CNS is a complex and structurally diverse organ that comprises multiple functional subdivisions. Neurons account for approximately half of the brain volume; the remainder consists of glial and vascular elements. In addition to mechanical support of neurons, the glia have important regulatory functions (eg, neurotransmitter handling and maintenance of the metabolic milieu of the neuropile) that, at present, are imperfectly understood.
Metabolic rates differ considerably within the brain tissue; for instance, there is an approximately fourfold difference in cerebral metabolic rate for oxygen (CMRO 2 ) and CBF between cortical gray matter and white matter. Flow and metabolism are coupled, and under physiologic conditions, including sedation and general anesthesia, this coupling is generally preserved ( Figs. 2.1 and 2.2 ). Intravenous anesthetic agents such as propofol seem to preserve flow-metabolism coupling better than volatile agents. In humans, this coupling is evident during anesthetic-induced electroencephalogram (EEG) burst suppression, as demonstrated by transcranial Doppler ultrasonography (TCD) studies during normothermia , and during mild-to-moderate hypothermic cardiopulmonary bypass.
Regulation of Cerebral Blood Flow
A precise regulatory system has evolved in the CNS whereby instantaneous increases in metabolic demand can be met by a local increase in CBF and substrate delivery. As has been known for a long time and demonstrated with multiple imaging modalities, the time course of this regulatory process is rapid. , Contralateral cortical areas manifest increased flow with hand movement, and a variety of motor and cognitive tasks can be mapped with CBF techniques. Visual stimulation results in almost immediate increases in flow velocity through the posterior cerebral arteries. Positron emission tomography (PET), magnetic resonance imaging (MRI) and time-resolved near-infrared spectroscopy (NIRS) are beginning to unravel the interrelated functions and their temporal relationships in various cortical areas activated by complex phenomena such as language and visual processing. As in most specialized vascular beds, this flow-metabolism coupling is critical during times of stress or extreme physiologic conditions, such as hypotension hypoxia and hypothermia. These pathologic processes engage regulatory mechanisms to keep flow at physiologic levels.
The term autoregulation is used by some to describe the hemodynamic response of flow to changes in perfusion pressure independent of flow-metabolism coupling. The problem with this approach is that the precise mechanisms responsible for maintenance of CBF are poorly understood. One could argue that autoregulation principally implies a matching of flow to metabolism, irrespective of the underlying mechanism. For example, the ability of the cerebral vasculature to dilate in response to tissue hypoxia certainly qualifies as an autoregulatory phenomenon, and it may be an oxygen-sensitive mechanism that regulates vascular resistance. Perhaps when the mediators of these “autoregulatory” events are more precisely known, better terminology can be devised. Autoregulatory responses are those that maintain the internal milieu of the CNS. Those that endanger CNS well-being are dysregulatory . Semantics aside, a clinical distinction can be made between two distinct processes that may or may not be mechanistically related—flow-metabolism coupling and active vasomotion in response to circulatory perturbation. There seems to be an elegant dichotomy of control in the cerebral vascular bed. The “distal vascular” bed can respond rapidly to the sudden changes in the metabolic needs of the tissue, whereas the “proximal vasculature” ensures adequate delivery of blood across a range of perfusion pressures. The two systems probably communicate with each other, in part through nonadrenergic, noncholinergic neurons that innervate the distal penetrating arterioles. ,
Since Roy and Sherrington put forth their hypothesis more than 100 years ago, the prevailing paradigm has been that local metabolic factors are involved in flow-metabolism coupling. However, pure changes in perfusion pressure undoubtedly involve a myogenic response in vascular smooth muscle as well (Bayliss effect). This myogenic response may actually consist of two separate mechanisms, one responding to mean blood pressure changes and the other sensitive to pulsatile pressure. Evidence shows that flow, independent of pressure, may affect vascular resistance. An overwhelming number of metabolic mediators for CBF regulation have been proposed, including hydrogen ion, potassium, adenosine, glycolytic intermediates, and phospholipid metabolites. , Both neurons and astrocytes seem to participate in flow-metabolism coupling. , Endothelium-derived factors such as nitric oxide (NO) enable the endothelium to function as a transducer that controls the tone of the vascular smooth muscles. The interactions between the endothelium and the smooth muscle cells are complex and have built in redundancy. Cellular mechanisms within the endothelium and the vascular smooth muscles often converge on intracellular Ca 2 + as their final common pathway. However, no single mechanism seems to play a preeminent role in regulating blood flow to the brain. ,
Independent assessment of CBF and oxygen utilization by means of PET reveals that the increase in brain activity in response to sensory stimulation results in a minimal increase in O 2 consumption (CMRO 2 , ~ 5%) but a considerably greater increase (~ 30% to 50%) in blood flow. Such an increase in CBF is coupled to the increase in the cerebral metabolic rate for glucose. The disproportionate increases in CBF and cerebral metabolic rate for glucose in comparison with CMRO 2 raise the possibility of anaerobic metabolism in the brain.
The issue of anaerobic metabolism in the brain has been debated ever since these observations were first made and conflicting evidence has been presented in this area. In support of anaerobic metabolism, evidence shows transient lactate production during photoptic stimulation. On the other hand, evidence of an early rapid increase in tissue deoxyhemoglobin concentrations during cortical activity suggests a rise in oxygen use. The temporal relationship between neuronal activation, glucose utilization, and blood flow coupling is still being debated. It is now believed that neuronal activation prompts immediate anaerobic glucose metabolism to meet the energy demands for glutamate release. However, clearance of glutamate requires oxidation of glucose in amounts that are in excess of oxygen utilization, resulting in a net efflux of lactate. Under physiologic conditions, lactate is subsequently oxidized to generate additional energy.
Perivascular innervation in the brain has been recognized since Willis first described the cerebral circulation in 1664. Nevertheless, the precise function of this innervation remains obscure. The current paradigm suggests that autonomic nerves are not necessary for regulatory responses but may modify them in several important ways. A major deficiency in the “local metabolic,” or “negative-feedback” theory is that the necessary temporal relationship between accumulation of vasoactive metabolites and flow increases has not been adequately demonstrated. In addition, in many situations, CBF and CMRO 2 change in the same direction but CBF increases out of proportion to metabolic rate, such as during seizure activity. There is mounting evidence that local neuronal and glial influences play a greater role in regulation of CBF than previously appreciated. Though the understanding of these mechanisms is still evolving, they may better explain the discrepancy seen between the magnitudes of increases in CBF and CMRO 2 .
Cellular Mechanisms of Cerebral Vasomotion
The remarkable ability of the cerebral vessels to respond to changes in cerebral metabolism, perfusion pressure, and milieu interior, such as Paco 2 , is mediated by a number of cellular mechanisms. These mechanisms involve nitric oxide, prostaglandins (PGE 2 , PGI 2 , and PGF 2α ), vasoactive peptides, potassium channels, and endothelin. , ,
Nitric Oxide
Although it is unlikely to be directly involved in pressure autoregulation itself, NO is the subject of intense scrutiny as a mediator of vascular tone and as a neurotransmitter. , The interest in NO results from the identification of the multiple biologic roles it plays as a messenger molecule. Although until recently no evidence had shown it to have any biologic function at all in vertebrates, NO now appears to have at least the following major roles: (1) bactericidal and tumoricidal effects in white blood cells, (2) a neurotransmitter, and (3) a moderator/mediator of vascular tone, functioning as an “endothelium-derived relaxing factor.”
NO is synthesized from l -arginine by nitric oxide synthase (NOS). There are at least three isoforms of NOS: endothelial (eNOS), neuronal (nNOS), and inducible (iNOS). Of these, eNOS and nNOS exist in the normal brain, whereas iNOS synthesis can be induced by endotoxins and cytokines. Endogenous inhibitors of NOS, such as asymmetric dimethyl- l -arginine (ADMA), are produced during protein catabolism and may reach concentrations sufficient to inhibit NOS activity in the brain. NO action has been studied through the use of arginine analogues such as NG-nitro- l -arginine methyl ester ( l -NAME), 7-nitroindazole, and aminoguanidine, which can nonselectively or selectively block NO synthesis. NO appears to influence basal tone, as well as the endothelium-dependent response to acetylcholine in cerebral arteries and vasogenic dilation from stimulation of nonadrenergic, noncholinergic nerves. In general, topical, systemic, and intra-arterial application of NO donors increases CBF in several animal species. , Intra-arterial injection of the NO donor nitroprusside into angiographically normal territories in patients with cerebral arteriovenous malformations failed to augment CBF. A similar failure of intra-arterial nitroprusside was seen in healthy primates. , In contrast, a study in human volunteers found that systemic and intra-arterial administration of NG-monomethyl- l -arginine ( l -NMMA), a nonspecific inhibitor of eNOS, decreases CBF. , The latter findings suggest that NO may be involved in regulation of basal cerebrovascular tone. After synthesis, NO diffuses into the vascular myocyte and activates guanylate cyclase, forming cyclic guanosine monophosphate (cGMP). A protein kinase is stimulated by cGMP, resulting in phosphorylation of the light chain of myosin and thus vascular relaxation. NO may also act partly through calcitonin gene-related peptide (CGRP) and ATP-sensitive potassium (KATP) channels. NO partly acts also by suppressing endothelial generation of vasoconstrictors such as thromboxane A 2 . In pathologic settings, such as vasospasm and hypoxia, Rho kinase, a serine threonine kinase, is emerging as a potent mediator of sustained vasoconstriction that in part acts through the NO pathway. Inhibition of Rho kinase increases cerebral blood flow. In middle cerebral artery occlusion models, Rho kinase inhibition improves neurologic outcome. , Rho kinase inhibition increases eNOS synthesis, and Rho kinase seems to negatively regulate eNOS activity. Calcium is intimately involved in vascular relaxation by NO. NO appears to be formed on demand and is not stored in vesicles—the traditional fate of neurotransmitters.
The role of NO in the vasodilitatory response due to changes in perfusion pressure or carbon dioxide (CO 2 ) remains to be coherently defined. For example, nonspecific inhibition of NOS in primates does not affect pressure autoregulation but impairs response to CO 2 . However, in humans, nonspecific inhibition of NOS results in a decrease in CBF but does not affect response to hypercapnia. In rodents, nonspecific inhibition of NOS impairs autoregulatory response to hypotension in basilar artery irrigation. While selective nNOS inhibition by 7-nitroindazole has no effect on baseline blood flow, 7-nitroindazole can prevent the increase in blood flow due to neural activation. In canines, 7-nitroindazole decreases collateral blood flow during middle cerebral artery occlusion.
Some investigators have reported that NO appears to play a role in dilation in response to CO 2 . In other experiments, however, its participation in hypocapnia-induced vasoconstriction could not be demonstrated. Iadecola and Zhang proposed that NO plays either an “obligatory” or a “permissive” role in CO 2 -induced vasodilation. Obligatory implies that NO directly mediates vasodilation through that mechanism. For example, topical application of glutamate agonists results in vasodilation that can be markedly attenuated by inhibition of NOS. Therefore, NO seems to play an obligatory role in glutamate-mediated vasodilation. Permissive implies that NO facilitates relaxation but near-complete inhibition of NOS only partly attenuates the vasodilator response. Because hypercapnic response is only partly attenuated by NOS inhibition, NO’s role is described as permissive, with other mechanisms also contributing to hypercapnic dilation. NO appears to play a much greater role in hypercapnic vasodilation in adults than in neonates. The site of action for CO 2 -induced NO production may not be in the endothelium but, rather, in the perivascular structures, such as astrocytes.
The participation of NO in hypoxia-induced vasodilation does not appear to be physiologically important. , , In regard to anesthetic effects on CBF, NO appears to interact with the cerebral vasodilatory effects of both halothane and isoflurane. The role of NO as a neurotransmitter undoubtedly will prove to be significant for care of the patient with neurologic disease through its interactions with anesthetic depth and cerebral ischemic states, , in particular the pathogenesis of vasospasm after subarachnoid hemorrhage (SAH). Inhibition of NO synthesis leads to vasoconstriction due to unopposed effects of endothelial prostanoids, such as thromboxane A 2 and prostaglandin F 2α . Vascular abnormalities in disease states that significantly predispose the brain to damage, such as diabetes mellitus, may also be related to an NO-mediated mechanism.
Vasoactive Peptides
In the cerebral circulation, perivascular nerves contain several vasodilator peptides, including CGRP, substance P, and neurokinin A. Vasodilation with CGRP, unlike with substance P and neurokinin A, is independent of endothelin. CGRP acts by increasing intracellular cyclic adenosine monophosphate (cAMP) concentrations and partly mediates cerebral vasodilation in response to hypotension, cortical spreading depression, and cerebral ischemia. Vasodilation by NO is in part mediated by CGRP. , CGRP probably does not play a role in vasodilator response to hypoxia or hypercapnia. The physiologic roles of substance P and neurokinin A are not yet understood. Substance P may mediate vasodilation during pathologic derangements such as cerebral and meningeal inflammation and edema.
Potassium Channels
Of the several potassium channels in the cerebral vessels, two are of particular importance in the regulation of vascular tone: KATP channel and calcium-activated potassium (KCa) channel. A third potassium channel, pH-sensitive delayed rectifier potassium channel, may play a role in hypercapnia. Opening of potassium channels triggers potassium efflux from the vascular smooth muscle cell, hyperpolarizes the cell membrane, closes the voltage-dependent calcium channels, decreases calcium entry into the cells, and ultimately relaxes the muscles. KATP channels are opened by a decrease in intracellular pH and are inhibited by an increase in intracellular ATP concentrations and by sulfonylureas. Activation of KATP channels may partly mediate vasodilation by acetylcholine, CGRP, or norepinephrine (noradrenaline). KATP channels may play some role in vasodilation during hypotension, hypercapnia, acidosis, and hypoxia. KCa channel-mediated vasodilation is due partly to astrocyte-derived carbon monoxide, which diffuses into the smooth muscle cells. Large-conductance KCa (BKCa) channels are the most important of the several KCa channels found in the cerebral circulation. These channels can be selectively blocked by tetraethylammonium, charybdotoxin, and iberiotoxin. Inhibition of BKCa channels results in cerebral vasoconstriction in the large arteries, suggesting that BKCa channels may be involved in the regulation of basal cerebrovascular tone in these vessels. BKCa channels are activated by cGMP, cyclic adenosine monophosphate, and NO and are partly responsible for hypoxia-induced vasodilation of cerebral arteries. , , ,
Prostaglandins
Prostaglandins such as PGE 2 and PGI 2 are vasodilators but thromboxane A 2 and PGF 2α are vasoconstrictors in the cerebral circulation. Synthesis of prostaglandin H 2 from membrane phospholipids involves two critical enzymes, phospholipase and cyclooxygenase. Prostaglandin H 2 is converted into other prostaglandins by subsequent enzymatic steps. Although cyclooxygenase can be inhibited by aspirin, naproxen, and indomethacin, only indomethacin impairs hypercapnic vasodilation in humans. ,
Prostaglandins probably play a more significant role in the regulation of CBF in neonates than in adults. Inhibition of phospholipase by quinacrine hydrochloride abolishes the cerebrovascular response to hypercapnia, and hypoxia in newborn animals. Endothelial damage and indomethacin also abolish hypercapnia-induced vasodilation and the increase in cerebrospinal fluid (CSF) PGI 2 concentrations. However, indomethacin-impaired CO 2 reactivity can be restored by very low concentrations of PGE 2 . This suggests that prostaglandins may not be direct mediators of hypercapnic vasodilation but that small amounts of prostaglandins are necessary for the CO 2 response to hypercapnia to occur and that prostaglandins thus play a so-called permissive role.
Endothelin
Endothelin is a vasoactive peptide that is synthesized by the brain and the vascular endothelium. There are three isoforms of endothelin. The brain synthesizes endothelin-1 (ET-1) and endothelin-3 (ET-3) but not endothelin-2 (ET-2). The vascular endothelium synthesizes ET-1. The two receptors for endothelin are endothelin A (ETA) and endothelin B (ETB). Activation of ETA receptors causes vasoconstriction, and activation of ETB receptors may cause either vascular relaxation or constriction. Vascular relaxation is thought to be mediated by endothelin receptors on the endothelium, whereas constriction is probably mediated by endothelin receptors located on the smooth muscle cells. ETA receptors are probably more sensitive to ET-1 and ET-2 than to ET-3. The ETB receptor is equally sensitive to all isoforms of endothelin. , Endothelin most likely acts through influx of extracellular calcium, which is probably mediated by protein kinases. The vascular smooth muscle contraction caused by endothelin is sustained, suggesting that endothelin is not involved in rapid adjustment of cerebrovascular resistance (CVR). Topical applications of endothelin receptor antagonists do not alter resting CVR. Endothelin has been implicated in vascular spasm after SAH. , In experimental models of SAH, ETA and ETB receptor antagonists prevent evolution vasospam. Endothelin-induced vasospasm can also be reversed nonspecifically by calcium channel blockade and seems to be more responsive to intra-arterial nicardipine than to verapamil. , Early results of clinical trials showed that intravenous infusion of an ETA receptor antagonist, clazosentan, resulted in a decrease in the incidence of vasospasm after SAH. Intravenous clazosentan infusion reduces the severity of the established cerebral vasospasm. However, despite improvements in angiographic vasospasm with ETA receptor antagonists, more recent clinical analyses have shown no improvement in vasospasm-related cerebral infarction, new cerebral infarction, or case-fatality. Furthermore, treatment with ETA receptor antagonists was associated with higher incidence of pulmonary complications, hypotension, and anemia. Thus, enthusiasm for using these agents has waned.
Anatomic Considerations
The primary arterial supply to the brain consists of the anterior circulation, which comprises the two carotid arteries and their derivations, and the posterior circulation, consisting of the two vertebral arteries, which join to form the basilar artery. Collateral arterial inflow channels are a cornerstone of CBF compensation during ischemia. The principal pathways are embodied in the circle of Willis. This hexagonal ring of vessels lies in the subarachnoid space and encircles the pituitary gland ( Fig. 2.3 ). In many patients the circle of Willis is incomplete. The primary routes of collateral circulation are the Willisian channels (anterior communicating artery [ACA] and posterior communicating artery [PCA]) and the ophthalmic artery via the external carotid artery. In a normal individual, there is probably no net flow through these communicating vessels but rather a to-and-fro movement of blood that maintains patency by preventing thrombosis and atresia. These vessels allow flow when a pressure differential develops. The second main recourse for collateral flow in the hemispheres is the surface connections between pial arteries that bridge major arterial territories (ACA-PCA, ACA–middle cerebral artery [MCA], MCA-PCA). These connections are called by various names. “Pial-to-pial anastomoses” or “collaterals” seem to be the most logical terms, but they are also called “leptomeningeal pathways.” These pathways may protect the so-called border zones or watershed areas between vascular territories. A considerable amount of confusion in terminology is found in this domain. Physiologically, a more precise term might be “equal pressure boundary,” that is, where, under normal circumstances, pial flow does not cross collateral pathways into an adjacent territory because the pressures on either side of this distal territorial boundary are equal. Considerable variation exists in the anatomic location of these boundaries, and they may change during the course of treatment, if the vascular architecture is altered, such as after multiple arteriovenous malformation (AVM) embolizations.
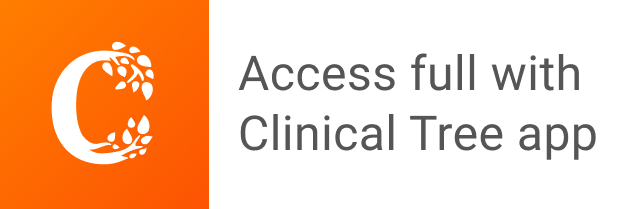