FIGURE 13.1 Positron emission tomography image of an intracerebral hemorrhage. Note central area of necrosis surrounded by area of decreased perfusion. (Courtesy of Michael Diringer, Washington University School of Medicine.)
New techniques of bolus tracking in both magnetic resonance imaging (MRI) and computed tomography (CT) have allowed for acute assessments of cerebral perfusion. These techniques are known as CT perfusion and MR perfusion. In these techniques, a bolus of a contrast agent is detected either through changes in T2 signal or Hounsefield units. Estimations of CBF can be made by measuring the transit time required for these boluses to pass through cerebral tissue. These radiologic modalities are being used widely for the acute assessments of cerebral infarctions (14,15).
Regulation of CBF by Oxygen
Under normal conditions, CBF and regional distribution of oxygen are tightly coupled. Increases in cerebral metabolism lead to an increase in the delivery of oxygen and glucose to metabolically active tissue. The cerebral metabolic rate of oxygen consumption (CMRO2) is the rate of oxygen utilization by cerebral tissue. It is calculated by the Fick method as the product of CBF and the cerebral oxygen arterial–venous difference of an inert nondiffusible substance. Direct and indirect methods exist to estimate both CBF and CMRO2. Cerebral oxygen delivery (CDO2) is the product of CBF and the oxygen-carrying capacity of hemoglobin. The normal mean capillary partial pressure of oxygen (PO2) is approximately 65 mmHg, representing a difference between normal arterial PO2 (PaO2 = 90–95 mmHg) and venous PO2 (PvO2 = 35–40 mmHg). Normal values for standard measures of CBF, CMRO2, and CDO2 are listed in Table 13.1.
TABLE 13.1 Normal Values for Cerebral Blood Flow and Metabolism | |
![]() |
CDO2 can also be affected by changes in the concentration of oxygen bound to hemoglobin. Hemoglobin has a high affinity for binding oxygen with greater than 90% of the hemoglobin binding sites for oxygen saturated at PaO2 greater than 70 mmHg; increasing PaO2 above this level, therefore, has little effect on oxygen delivery.
At PaO2 below 50 mmHg, there is a rapid increase in CBF (Fig. 13.2). This is more significant in grey, as opposed to white, matter. There is a linear relationship between the arterial oxygen content and CBF in hypoxic states; however, the relationship between CBF and the PaO2 is curvilinear (16). Vasoactive effects may be caused directly by oxygen or mediated though adenosine A2 receptors (9). Several other mechanisms exist to maintain oxygen delivery to the brain. Cerebral hypoperfusion will lead to depolarization of medullary neurons controlling sympathetic output. This results in a compensatory increase in blood pressure and heart rate. Decreased cerebral perfusion leading to decreased arterial oxygen delivery to the cerebral capillary bed will lead to venodilation, lowering the postcapillary pressure and increasing flow across the capillary bed. Extraction of oxygen bound to hemoglobin is increased across the capillary bed as CBF continues to decrease. This increase in oxygen extraction can be detected by measuring oxygen concentration in jugular venous blood and comparing it to arterial oxygen content. Finally, increases in local hydrogen ion concentration will occur as ischemia develops, presumably due to lactate production from anaerobic metabolism. The resultant shift in the oxygen displacement curve of hemoglobin to the right will result in increased oxygen release from hemoglobin to the local cerebral tissue.

FIGURE 13.2 Alterations of regional cerebral blood flow (rCBF) to changes in arterial oxygen tension. Note oxygen tensions below approximately 50 mmHg lead to a sharp increase in rCBF. (From Golanov EV, Reisw DJ. Oxygen and cerebral blood flow. In: Welch KMA, Caplan LR, Reis DJ, et al., eds. Primer on Cerebrovascular Disease. San Diego, CA: Academic Press; 1997:58–60.)
Regulation of CBF by CO2
Changes in the partial pressure of carbon dioxide (PCO2) have significant effects on CBF. One mmHg change in PaCO2 can result in an approximately 2.5% to 4% change in CBF. This effect is more pronounced in the grey matter than in the white matter. The response curve of PaCO2 is sigmoidal, with the CBF response flattening below 15 to 20 mmHg and above 100 mmHg. Vasodilation occurs at all vessel sizes, but is most pronounced on the smaller arterioles (9).
Changes in vessel diameter are mediated through alterations in cerebrospinal fluid hydrogen ion concentrations (CSF pH). The direct application of CO2 or bicarbonate to pial arterioles does not affect vessel diameters. Since CO2 is freely diffusible across the blood–brain barrier, changes in PaCO2 will affect both CSF hydrogen ion and bicarbonate concentrations (17). The response of changes in hydrogen ion concentration is relatively short-lived, lasting only a few hours as the choroid plexus of the brain will compensate for changes in hydrogen ion concentration by adjusting the production of cerebrospinal fluid bicarbonate (18).
CEREBRAL AUTOREGULATION
Cerebral autoregulation refers to the capacity of CBF to remain constant despite changes in cerebral perfusion pressure (CPP). This intrinsic control allows for relative constant CBF over a wide range of CPP. The shape of the autoregulatory curve can be modified by a number of extrinsic factors: hypertension, hypocarbia, and increased sympathetic nerve activity will increase both the upper and lower ranges of autoregulation. Chronic hypotension, hypercarbia, and parasympathetic activity will lower the set points of autoregulation. The autoregulatory curve will shift upwards with advancing age. In the healthy, normotensive individual, CBF is relatively constant between CPP (or mean arterial pressure [MAP]) of 50 to 150 mmHg. Outside this range, CBF has a linear correlation with CPP, otherwise described as a pressure-passive phenomenon (Fig. 13.3) (18,19).
In most vascular beds, autoregulation occurs at the level of the arterioles. However, a significant proportion of vascular resistance in the cerebral circulation is modulated at the level of the cerebral arteries. In cats, dogs, and monkeys, approximately 40% of the cerebral vascular resistance is mediated by changes in the baseline pial artery diameter of vessels greater than 400 μm in diameter (19).
In response to hypotension, both cerebral arteries and arterioles will dilate to maintain constant CBF. Vessel dilation progresses from the largest vessels to the smallest in response to decreases in CPP (16). Cerebral arterioles will also continue to dilate below the lower limit of autoregulation (20). Further drops in pressure slow flow across the capillary bed and lead to an increase in the oxygen extraction coefficient from hemoglobin (21); continued hypotension progresses to ischemia. Oxygen deprivation leading to neuronal cell death proceeds through several distinctive steps. The high metabolic activity of neurons rapidly depletes oxygen-derived ATP and phosphocreatine stores, leading to failure of synaptic transmission. The EEG at this point becomes flat and consciousness is lost. Electrical failure occurs as CBF falls below 16 to 20 mL/100 g tissue/min and cerebral oxygen consumption falls below a third of its normal resting metabolism. Restoration of CBF after electrical failure, however, will lead to functional recovery of the cell. Further decreases in CBF to less than 10 mL/100 g/min will lead to energy failure needed to maintain the activity of the membrane sodium–potassium pump. As flow continues to decrease, membrane depolarizations occur and ionic gradients are lost as potassium effluxes out of the, and sodium and water enter, the cell, leading to the development of cytotoxic edema (Fig. 13.4).

FIGURE 13.3 Cerebral autoregulatory curve with cerebral blood flow remaining constant between cerebral perfusion pressures (CPP) of 50 and 150 mmHg (solid line). Superimposed curve of cerebral blood volume (CBV) at variable CPP (dotted line). The top portion of the figure illustrates the cerebral arteriolar caliber. Note that at CPP below the level of cerebral autoregulation, arteries and arterioles begin to collapse (passive collapse). CBV decreases from a point of maximal dilation seen near the lower end of CA (vasodilatory cascade zone) to a constant blood volume (zone of normal autoregulation). Higher CPP leads to autoregulatory breakthrough and parallel increases in both CBF and CBV (autoregulatory breakthrough zone). ICP, intracranial pressure. (From Rose JC, Mayer SA. Optimizing blood pressure in neurological emergencies. Neurocrit Care. 2004;1:287–299.)
In a similar fashion, cerebral pial arteries and arterioles will constrict as CPP (or MAP) increases. Maximal vasoconstriction occurs at CPP (MAP) of 160 to 170 mmHg. Above these pressures, passive dilation of the blood vessels occur with resultant autoregulatory failure, breakdown of blood–brain barrier, cerebral hyperperfusion, and development of vasogenic edema.
Several theories have been proposed to explain the mechanisms of cerebral autoregulation. These can be broadly categorized into myogenic, metabolic, and neurogenic (21).
The myogenic theory postulates that smooth muscle cells of the cerebral arteries and arterioles constrict or dilate in response to the transmural pressure that is generated across the vessel wall (22). This may be mediated by alterations in the position of the actin myosin filament in the vessel wall (23). Another theory is that changes in vessel wall shear stress induced by alterations in blood flow induce the release of vasoactive substances from the vascular endothelium that subsequently leads to vessel wall constriction or dilation (24). The myogenic theory is supported by the rapidity by which cerebral autoregulation occurs. However, the myogenic theory by itself cannot account for all the regulation that occurs. In jugular venous hypertension, intravascular pressure is increased. This condition should lead to arterial vasoconstriction; however, vasodilation is most commonly found under these circumstances (25). It has, therefore, postulated that myogenic factors may play a significant role only when metabolic factors are not significant. Alternately myogenic factors may work synergistically with metabolic factors providing changes in vascular tone required to optimize the metabolic response (26).

FIGURE 13.4 Blood flow thresholds for cerebral function and metabolism. As blood flow decreases, synaptic transmission and electrical activity ceases (electroencephalographic silence). Further decreases in flow lead to loss of ionic homeostasis and cell death. The ischemic penumbra describes brain tissue with blood flows below electrical failure but above membrane failure. CBF, cerebral blood flow; EEG, electroencephalogram; ATP, adenosine triphosphate. (From Veremakis CV, Lindner DH. Central nervous system injury: essential physiologic and therapeutic concerns. In: Civetta JM, Taylor RW, Kirby RR, eds. Critical Care. Philadelphia, PA: Lippincott–Raven Publishers; 1997:273–289.)
The metabolic theory of pressure autoregulation postulates that the local changes in CBF found with changes in cerebral metabolic activity are mediated through the releases of local neurochemical substances from the nonvascular cells of the central nervous system (21). The tight coupling of flow to metabolism, and the timing of autoregulation support this postulated mechanism. Several substances have been implicated, including hydrogen ion, carbon dioxide, nitric oxide (NO), adenosine, potassium, and calcium (27). Experimental evidence exists in favor and against all of the postulated mediators (21,28–30); varying combinations have also been suggested. Most recently, changes in potassium levels mediated through alterations in calcitonin gene-related peptides have been suggested to be a mechanism of arteriolar dilation (31). Observations that changes in CPP alter the degree of endothelial oxygen tension suggest that this may be another important mechanism for autoregulation (32).
The direct role of neurogenic mechanisms on cerebral autoregulation is probably limited. The sympathetic and parasympathetic nervous systems play a role in modulating the cerebral autoregulatory curve, but direct neural control over small arteries or arterioles regulating focal changes in CBF does not occur. Other neurogenic mediators may be in a position to regulate vascular tone (33). The localization and central control of these nerves have so far proved elusive (21,34).
Measurement of Autoregulation
Severe TBI, infarcts, hemorrhagic strokes, and space-occupying lesions can result in impairment of cerebral autoregulation. As boundaries of autoregulation are exceeded, either hypoperfusion or hyperperfusion can occur, resulting in irreversible injury.
The index of autoregulation is calculated by a correlation coefficient between two factors within a time series. The coefficient ranges from −1 to +1 where a negative correlation is effective autoregulation and a positive correlation is impaired autoregulation. Several techniques utilized to calculate autoregulation indices include transcranial Doppler (TCD), intracranial pressure (ICP), partial pressure of brain oxygen (PbtO2), and near-infrared spectroscopy (NIRS). Each of these techniques is surrogates of CBF and offer slightly different indices of autoregulation. Whether guiding therapy based on the status of autoregulation can improve functional outcomes in patients with critical brain injury remains to be elucidated.
ICP Waveform
The classical variations of ICP waveforms include pulse and respiratory components. The waves of ICP correlates with every pulse and represent large cerebral vessel pulsations. The pulse wave is made of three points: P1, P2, and P3. P1, otherwise known as the percussive wave, is produced by the direct transmission of the choroid plexus arterial pulsation; P2, also known as the tidal wave, represents brain compliance. In states of poor cerebral compliance, such as brain trauma, the P2 peak can be pathologically higher than the P1 peak. Closure of the aortic valve produces P3, the dicrotic wave which was previously thought to originate from the venous system. As ICP increases, all three components of the waveform increase in amplitude. However, as ICP continues to increase, P2 dominates and the waveform assumes a rounded appearance. Hypocapnia, on the other hand, will result in constriction of cerebral blood vessels and the amplitudes of all three P waves will be diminished.
ICP Dynamics
Normal ICP is usually between 5 and 8 mmHg, but can be as high as 15 mmHg in some circumstances. Beyond this, some degree of intracranial pathology should be suspected (35). Normal ICP fluctuates rhythmically approximately 3 to 5 mmHg. The sinusoidal pattern can be seen on an ICP pressure trace and was originally described by Traube and Hering (36). The origin of these waves is unknown, but may have to do with phasic constriction and dilation of the cerebral arterioles (37) or to changes in intrathoracic pressure during the phases of the respiratory cycle; as ICP increases, this variation is lost (38).
The ICP–volume curve demonstrates a relatively flat portion where increases in volume are accommodated with little change in pressure. At some inflection point, these processes are exhausted and small changes in volume lead to larger increases in ICP (Fig. 13.3). This pressure–volume curve may, therefore, represent the displacement of various fluids from the intracranial space.
The approximate contents of the intracranial cavity consist of brain parenchyma (75%), blood (20%), and CSF (5%). Most of the intracranial blood resides in the venous side of the circulation (35). According to the Monroe doctrine, the overall volume of the contents of the intracranial cavity must remain constant. Thus, any increase in the intracranial contents from venous engorgement, intracerebral hemorrhage, tumors, edema, etc. must be compensated by an equal displacement of fluids or tissue. The flat portion of the ICP–volume curve represents displacement of CSF into the more compliant spinal subarachnoid space. Progressive increases in volume lead to further displacement of venous and arterial blood. Finally, brain tissue is displaced and herniation occurs (Fig. 13.5) (39).
The ICP–volume curve can be affected by the speed and duration of changes that occur in the intracranial cavity. For instance, tumors can grow to large volumes before becoming problematic because the slow-growing process allows for a gradual increase in intracranial compliance. Conversely, an acute hemorrhage of the same size will not be tolerated.
Intracranial compliance can be determined by measuring the change in ICP to a given volume. A volume–pressure response (VPR) is estimated by measuring the ICP response to an infusion of sterile saline through an intraventricular catheter (40). Small changes in response to this increased volume suggest that the patient is on the flat portion of the volume–pressure curve. Increases in ICP greater than 4 mmHg in response to 1 mL of fluid would suggest that intracranial reserve is limited. A more widely used index to measure intracranial compliance has been the pressure–volume index (PVI); this index estimates the volume needed to increase ICP by a factor of 10 (41).
Spontaneous and sustained elevations in ICP were noted by Lundberg early in the history of study of cerebral hemodynamics (42). The origin of these “plateau waves” (or A waves) has been speculative until the 1980s when Rosner and colleagues observed that plateau waves were always preceded by drops in CPP. The decreases in CPP are largely a result of decreases in MAP, but could also be due to excess CSF volume, hypercarbia, or changes in vascular resistance induced by metabolic factors (43). Vasodilation in response to the drop in CPP would be responsible for the sustained rise in ICP (i.e., the generation of the plateau wave).
CPP Dynamics
CPP is defined as the pressure difference between MAP and mean jugular venous pressure. However, when ICP is greater than jugular venous pressure, ICP is substituted for jugular venous pressure. Thus, CPP is typically reported as (18):
CPP = MAP − ICP
In healthy controls, ICP is less than 5 mmHg and so CPP and MAP are nearly equal.
As previously noted, decreases in CPP will lead to an autoregulatory cerebral vasodilation increase in CBV. In the setting of altered intracranial compliance, ICP can increase with rising CBV. This increase in ICP in turn compromises CPP in a feedforward loop. The process is spontaneously reversed by an acute elevation of blood pressure—a phenomenon known as Cushing response. This sympathetic response probably occurs because of oligemia affecting the brainstem centers modulating sympathetic activity (44).
MECHANISMS OF BRAIN INJURY
Immediate Concerns
The most important features in managing acute neurologic injury are management of the airway, oxygenation, and circulation issues, careful and repeated monitoring of vital parameters, and neurologic status. Patients should be rapidly transported to a trauma center or stroke center for prompt head imaging with immediate medical or surgical management of expanding mass lesions (45).

FIGURE 13.5 Three forms of the intracranial pressure volumes curves. The curve on the left represents the traditional teaching that compensatory mechanisms allow for small changes in pressure as intracranial volume increases. The middle section suggests that pressure may be better defined as the force per unit area needed to displace a certain volume of the intracranial contents. The last section suggests that the traditional pressure–volume curve actually represents superimposed displacement curves of varying substances in the cranial cavity. At low pressures (flat portion), cerebral spinal fluid is displaced downward into the compliant spinal subarachnoid space. As pressure increases, venous and arterial blood are displaced before brain parenchyma is displaced at very high pressures (cerebral herniation). CSF, cerebrospinal fluid. (From Rosner MJ. Pathophysiology and management of increased intracranial pressure. In: Andrews BT, ed. Neurosurgical Intensive Care. New York, NY: McGraw-Hill; 1993:57–112.)
The mechanisms of primary neurologic injury will vary depending upon the nature of the insult, but all will include, to some degree, the risk of secondary injury from cerebral hypoxia and ischemia. In head trauma, shearing injury develops due to different deceleration rates of grey and white matter. The resultant disruption of neurologic tracts is followed by a period of secondary ischemia. Prolonged seizure activity in status epilepticus (SE) leads to hippocampal ischemia, cell death and atrophy (46); a zone of ischemia surrounds all areas of cerebral infarction and cerebral hemorrhage (47).
The primary goal of acute neurologic management is to prevent secondary injury. This is attained by initiating measures to support CDO2 and reduce cerebral metabolic demands. Hypoxemia (SpO2 < 90%), hypotension (generally systolic blood pressure < 90 mmHg), expanding mass lesions, persistent seizures, and intracranial hypertension all potentially worsen secondary injury by limiting CDO2 and increasing cerebral metabolism. Immediate attention and correction of these problems can have a significant impact on both immediate and long-term outcome (45–47). A sense of urgency in the treating team is critical to provide early and aggressive resuscitative efforts (47).
The specific management of acute neurologic emergencies will vary according to the nature of the illness or injury; however, some general concepts can be applied to all neurologic emergencies. The basics of all life-support protocols focus on securing an adequate airway, restoring effective ventilation, and ensuring appropriate circulation. Loss of pharyngeal tone can lead to airway obstruction in patients with a depressed level of consciousness. Impairment of respiratory drive can occur after seizures, head trauma, anoxic injury, stroke, or metabolic disturbances. Decreases in cerebral perfusion are common after head or multisystem injury, shock, sepsis, or hemorrhage.
The acute management of neurologic injury must focus on the maintenance of CDO2. To accomplish this goal, adequate oxygenation, respiration, and blood pressure must be assured. Airway control via endotracheal intubation (ETI) for the neurologic patient should be performed immediately in all patients with a Glasgow coma scale (GCS) sum score of ≤8. Supplemental oxygenation and, in patients with severe anemia, red blood cell transfusions—to a hemoglobin of 8 to 9 g/dL—should be given to provide adequate oxygenation. The role of glycemic control and temperature modulation will be discussed later.
Once the basics of life support have been secured, a rapid history should be obtained from supporting personnel or family since in many circumstances the patient will be unable to provide a history. The immediate details surrounding the incident are crucial to understanding the nature of the injury.
A general physical examination prior to the neurologic assessment should focus on possible trauma or other medical conditions. Raccoon eyes or a Battle’s sign—bruising of the orbits and mastoid region, respectively—is evidence for a basilar skull fracture. In head trauma with a loss of consciousness, neck injury should be assumed and cervical stabilization should be used. A funduscopic examination may reveal papilledema or subhyaloid hemorrhages. Body or breath odors may suggest intoxication. New onset atrial fibrillation may be the only clue to the mechanism of a cerebral infarct. Subtle eye, finger, or limb movements may be the only signs of subclinical seizure activity.
A rapid neurologic assessment focusing on the level of consciousness, the cranial nerve examination, and any localizing features can be obtained within a few minutes. The GCS score is commonly used as a quantitative assessment of neurologic function (48). A new coma score, known as the FOUR score, has been developed and validated for application in the emergency room, medical intensive care unit, and the neurointensive care unit (Fig. 13.6) (49–52). The FOUR score has advantages over the GCS, especially in intubated patients as a third of the GCS score comprised of verbal responses. Moreover, the FOUR score identifies brainstem injury, locked-in syndrome, uncal herniation, and includes an assessment of the respiratory drive (49).
Emergent head imaging must be obtained as soon as the patient is considered hemodynamically stable to leave the emergency department or ICU. CT is the usual initial choice due to accessibility. A head CT without contrast is the best test to assess both for skull or bone fractures and for the presence of acute intracranial blood. Large intracerebral, epidural, or subdural hematomas may need emergent evacuation. New imaging techniques, such as CT perfusion and MRI perfusion–diffusion imaging, may be able to identify specific salvageable ischemic brain regions that are risk for infarction. Baseline laboratory studies to be assessed include serum glucose, electrolytes, complete blood count, prothrombin and activated partial prothrombin times, liver function tests, blood urea nitrogen, and creatinine levels.
The Brain Trauma Foundation guidelines recommends ICP monitoring for patients with severe head injury (defined as a GCS ≤ 8) and an abnormal CT scan. ICP monitoring is additionally recommended for severe head trauma and a normal CT scan if two or more of the following are present: abnormal motor posturing, age > 40 years, and systolic blood pressure < 90 mmHg (53). The practical value of other invasive monitoring modalities—such as local brain tissue oxygen monitoring—is less certain.
Specific treatment can be initiated once cardiopulmonary status has been stabilized and a general and neurologic assessment has been completed. Guidelines and protocols currently exist for prehospital management of head trauma, intravenous and intra-arterial lysis of cerebral thrombosis, intracerebral hemorrhage, and SE (45–47,54).
Cerebral Edema
Intracranial hypertension can be caused by expanding masses, cerebral engorgement, or the development of cerebral edema. Cerebral edema is roughly defined as an increase in brain tissue water and sodium of the extravascular space (10). Cerebral edema may compress brain structures, leading to herniation syndromes or reduce cerebral perfusion with subsequent infarction (55).
Cerebral edema can also be defined according to its location or mechanism of production. Edema can occur either inside the cells of the brain (intracellular) or outside the cells and the intravascular space in the interstitium (interstitial). While certain forms of cerebral edema may predominate, pure forms of either type of edema rarely exist. Cytotoxic edema is the term employed to describe the intracellular edema that develops after the loss of cell wall integrity (56). The terminology implies a toxic etiology but it is most often seen in cellular energy failure due to ischemia or hypoxia. Vasogenic edema represents an expansion of the interstitium due to disruption of the capillary blood–brain barrier, which allows extravasation of fluid from the intravascular space. Interstitial edema develops secondary to increases in the hydrostatic pressures of the ventricular system draining the CSF. Osmotic edema refers to the intracellular swelling that occurs due to rapid changes in brain sodium concentrations or due to osmotic dysequilibrium syndromes (10).

FIGURE 13.6 Instructions for the assessment of the individual categories of the FOUR score. (From Wijdicks EFM, Bamlet WR, Maramattom BV, et al. Validation of a new coma scale: the FOUR score. Ann Neurol. 2005;58:585–593. © 2007. Mayo Foundation for Medical Education and Research.)
Hypoxic Ischemic Injury
Cerebral ischemia develops if cerebral oxygen utilization cannot meet metabolic demands. This can result from problems with CDO2, increased metabolic demands, or impaired oxygen utilization. Decreases in CDO2 can occur with decreases in CBF due to stroke, increased ICP, decreased cardiac output, or hypotension. Cerebral metabolic rate is increased with increased cerebral activity, seizures, or hyperthermia; blood loss or carbon monoxide poisoning can decrease oxygen-carrying capacity (57).
Cerebral ischemia can be categorized into focal and global causes. Focal ischemia occurs when there is severe or complete reduction of blood flow to one of the major arteries of the brain. Neurologic impairment develops in functional patterns attributable to the particular arterial distribution that is involved. This is caused most commonly by embolic or atherosclerotic large vessel occlusion. Global ischemia refers to severe reductions or cessation of blood flow to the entire brain. The most frequent cause is cardiac arrest, but can also be seen in any condition that leads to global cerebral hypoperfusion or hypoxia (2,57).
The various cellular components of the brain tissue exhibit selective vulnerability to ischemia. Neurons are most susceptible to cerebral ischemia followed by oligodendroglia, astrocytes, and endothelial cells. Specific neuronal populations also exhibit selective vulnerability to ischemia and hypoxia. The most susceptible neurons to anoxia are the CA1 and CA3 cell populations located in the medial hippocampus. These cells are the most widely connected and have the highest resting metabolic rate of all neurons. Similarly, highly metabolically active cells with high susceptibility to ischemia include the cerebellar Purkinje cells, cortical cell levels 3 and 5, and the medium-size neurons of the striatum (2,58).
Patterns of neuronal injury encountered with cerebral anoxia can be attributed to the selective ischemic vulnerability of varying cerebral cell types coupled with the different mechanisms by which ischemia or hypoxia can occur. Watershed or border-zone infarctions occur at the boundary between the perfusion territories of the large cerebral arteries. Cardiac, septic, and hemorrhagic shock are the most common etiologies for this pattern. Dysmyelination of the central white matter can develop in the setting of hypotension where hypoxia does not occur. Cerebral white matter is believed to be selectively vulnerable to this condition due to its decreased resting CBF compared to the more metabolically active grey matter (2).
Neuronal cell death is a product of both the severity and duration of ischemia. Thus, incomplete degrees of ischemia can be tolerated for longer periods of time. However, there is some critical threshold of ischemia which will ultimately lead to necrosis. The impact of ischemia can be modified by metabolic factors, such as hyperglycemia, temperature, and metabolic activity.
Critical reductions in CBF and cerebral oxygenation cause widespread failure of ionic pumps in cellular membranes, with efflux of potassium and influx of sodium and water causing cellular (cytotoxic) edema. Release of excessive amounts of glutamate is believed to mediate the process of excitatory cell death after ischemia. Normally glutamate, an excitatory neurotransmitter, is released into the synaptic cleft and rapidly cleared by energy-dependent cellular uptake mechanisms. In the setting of energy failure, extracellular glutamate levels increase. Most glutamate neurotoxicity is mediated through N-methyl-D-aspartate (NMDA) receptors. Stimulation of these receptors activates calcium channels that mediate calcium entry into the cell. Intracellular calcium subsequently activates a number of destructive enzymatic processes, including protease destruction of structural proteins, phospholipase destruction of plasma membranes with release of arachidonic acid and endonucleases capable of fragmenting DNA repair mechanisms. Mitochondrial uptake of calcium leads to interruption of electron transport and the development of oxygen free radicals (57,59,60).
The neuropathologic manifestations of cerebral ischemia are necrosis and apoptosis. Necrosis is characterized by cellular swelling, membrane wall lysis, and a resultant inflammatory reaction to clear the necrotic tissue; cells die in groups leaving large areas of necrotic tissue (61). Apoptosis is an organized and regulated form of cell death where intracellular and extracellular signals lead to a programmed process of cell death with preservation of the mitochondria. Pathologically, apoptosis leads to cell shrinkage, chromatin condensation, and dissolution of the cell membrane. Inflammation is not commonly seen.
In focal ischemia and infarction, a central region of necrosis can be surrounded by a potentially viable area of tissue described as the ischemic penumbra. The ischemic penumbra can be defined through CBF measures, electrophysiology, or biochemical and genetic methods. The penumbra is tissue that is potentially salvageable if circulation is restored.
Hyperthermia
There is a large body of evidence from experiments in laboratory animal models of cerebral ischemia showing that increases in brain temperature worsen brain injury (62); excitotoxicity is believed to be the most likely mechanism. Hyperthermia increases both glutamate release and extracellular concentration. Free radical production is accelerated and the sensitivity of neurons to excitotoxic injury is increased (63). Other postulated mechanisms for neurologic damage with hyperthermia include inhibition of protein kinases responsible for synaptic transmission and cellular repair, and the release of neuronal proteases. The latter is believed to be the mechanism for worsening cerebral edema at higher brain temperatures (64–67).
Hyperthermia worsens outcomes in ischemic stroke patients (68) with a 2.2-fold increase in morbidity and mortality for every 1-degree increase in temperature above 37.5°C (69). Similarly, these results have been extended to a neurologic intensive care unit population; hyperthermia in this population both worsened outcome and increased the length of stay (70).
Hyperglycemia
Hyperglycemia has been well documented to increase the infarct size and worsen outcome in ischemic stroke (71,72). More recent studies in patients treated with thrombolytics have supported these observations (73–76), although the negative effects of hyperglycemia may be limited to large vessel ischemia or occlusions (77). Hyperglycemia has also been associated with worse outcome in subarachnoid hemorrhage (SAH) (78); results in head trauma have been inconsistent (79,80).
Hyperglycemia in acute neurologic injury may be attributed to several different mechanisms. The most common proposed mechanism for the development of hyperglycemia is a hormonally induced stress response caused by the neurologic injury leading to an increase in catecholamine and cortisol release. Other proposed means by which hyperglycemia could occur in neurologic injury include uncovering of latent diabetes (72). Hyperglycemia may increase neurologic injury through a number of mechanisms. These include expansion of infarct size due to an associated reduction in perfusion (81), increases in cerebral metabolism (82) and cerebral edema (83), and potentiated calcium entry into neurons (84). The most widely supported mechanism of hyperglycemia-induced neuronal injury is through the production of free radicals causing oxidative stress and inflammation (85,86).
Insulin reduces ischemic brain damage in animal models of acute ischemia (87), perhaps through a direct neuroprotective role of insulin beyond the modulation of glucose levels (88). Insulin suppresses mediators of inflammation and coagulation, and increases endothelial NO release. The release of local NO could potentially lead to increased vasodilation and perfusion of the ischemic penumbra surrounding the core of infarcted tissue (89). A large (n = 1,400 subjects) multicenter insulin trial in ischemic stroke is currently underway, scheduled to complete in July 2018 (ClinicalTrials.gov Identifier: NCT01369069).
Hypoglycemia
Intensive glucose control comes at a cost of inadvertent hypoglycemia. An increase in mortality can be seen in even single episodes of severe hypoglycemia in ICU patients (90). Patients with glucose levels below 81 mg/dL (4.5 mMol/L) have been shown to have a higher mortality than patients with normal glucose levels (25.9% vs. 19.7%; OR, 1.42) (91). Factors such as CBF, and duration and degree of hypoglycemia can impact the degree of injury resulting from hypoglycemia. Neurotoxicity from hypoglycemia occurs as a result of oxygen free radical accumulation, activation of apoptotic pathways, and glutamate-mediated excitotoxicity (92). Studies with microdialysis and PET reveal increased glucose utilization in severe TBI patients (93) which would suggest against the need to maintain strict normoglycemia.
Blood Pressure
Hypertension is common after neurologic injury. The etiology is often multifactorial and may include underlying hypertension, catecholamine release to pain and stress, direct hypothalamic damage, or a physiologic response to volume depletion. In addition, cerebral autoregulation is disturbed to varying degrees after brain injury. Complete loss of autoregulation leads to CBF directly correlating with MAP. Disturbances in cerebral autoregulation can be global or focal, involving only areas adjacent to damaged brain.
The question whether the hypertension encountered during neurologic injury is pathologic or a normal compensatory and protective physiologic response has important therapeutic implications. In areas surrounding neurologic injury, the blood–brain barrier is often damaged. In these areas hypertension can lead to the development of cerebral edema by increasing the intravascular hydrostatic forces driving fluid into the interstitium of the brain. Brain area surrounding tumors, arteriovascular malformations, or local areas of trauma or infarction are particularly susceptible. Blood pressure management is often titrated to maintain systolic pressures between 110 and 140 mmHg after surgery to avoid the complications of postoperative edema and breakthrough hemorrhage. In severe carotid stenosis, the CPP to the affected cerebral hemisphere may be compromised leading to a shift of the cerebral autoregulatory curve downward. Postcarotid endarterectomy hypertension needs to be treated to avoid reperfusion injury (breakthrough hyperemia and hemorrhage). Reperfusion injury is also the main cause of hemorrhage after acute recanalization in ischemic stroke; thus, strict avoidance of severe hypertension is mandated in these patients.
Alternatively, over-aggressive management of hypertension after neurologic injury can be potentially deleterious. Brain areas with disturbed autoregulation may require a certain pressure to maintain adequate perfusion. An example of this is the development of plateau waves after head trauma produced by cerebral vasodilation in response to inadequate cerebral perfusion. Cerebral vasospasm after SAH is treated with induced hypertension. In selected small case series, induced hypertension has been used in the treatment of acute ischemic stroke (94). Optimal blood pressure should, therefore, to be titrated to the individual patient and disease process.
Cardiac Stunning
Cardiac stunning and neurogenic pulmonary edema can occur after acute neurologic catastrophes. This is most commonly seen after severe head trauma, SAH, SE, or intracerebral hemorrhage. The mechanism of cardiopulmonary damage is believed to occur through massive catecholamine release mediated by the sympathetic nervous system (95), the so-called Takotsubo cardiomyopathy (apical ballooning syndrome) (96). Studies on dogs revealed greater density of β-receptors in the apical myocardium as compared to the base (97); with a catecholamine surge, the adrenergic receptors become stunned resulting in apical ballooning.
Sympathetic innervation of the heart parallels the cardiac conduction system, which probably accounts for the noted electrocardiographic changes that can occur with severe neurologic injury. Deep T-wave inversions are usually reported as “cerebral T waves”; however, the spectrum of sympathetically induced electrocardiographic changes is broad and includes ST-elevations and depressions (102). Pathologically, cardiac contraction bands are seen surrounding the entry zone of the sympathetic endplates into the myocardium. These bands represent reperfusion injury from ischemic cardiac muscle. The muscle dies in a hypercontracted state, which ultimately becomes calcified (98). Cardiac enzymes are elevated, but this finding does not necessarily implicate coronary artery disease. Clinically, myocardial contraction is impaired; cardiac output and ejection fractions are decreased; and pulmonary edema is common and may be cardiogenic, neurogenic, or both.
Serial echocardiography indicates that cardiac function usually improves over a course of 1 to 3 weeks. However, the diagnosis of catecholamine-induced cardiac stunning implies a more severe neurologic injury, complicates medical management, and usually portends a worse outcome (99).
Neurogenic Pulmonary Edema
In neurogenic pulmonary edema, sympathetic-mediated pulmonary venoconstriction is believed to create the excessive hydrostatic pressure necessary to develop pulmonary edema (100). The sympathetic nervous system also innervates contractile elements of the pulmonary endothelial cells; catecholamine-mediated contraction of these cells can lead to opening of the tight junctions of the capillaries, allowing protein to flux into the pulmonary parenchyma (101). The process is self-limited and positive pressure ventilation, often combined with induced diuresis, is typically adequate to improve oxygenation.
Sodium Metabolism and Homeostasis
Disturbances in sodium metabolism and homeostasis are found in a variety of neurologic diseases. Circulating levels of antidiuretic hormone (ADH) are elevated in a number of acute neurologic emergencies, secondary to a catecholamine-induced stress response. This raises the question of whether the hyponatremia encountered is truly resulting from inappropriate ADH release or just represents an appropriate but excessive response.
Hyponatremia can have significant implications for the management of the neurologic patient. Acute hyponatremia leads to the development of intracellular edema with expansion of the size of the neuronal cell body. Unlike other cells in our body, the neuron needs to maintain its cell size and integrity in order to transmit electrical signals. The cellular response of the neuron to intracellular edema is to extrude intracellular osmoles to reduce the intracellular osmolality and return the cell size to normal (102); thus, chronic hyponatremia can be tolerated well. However, cellular swelling in response to severe acute hyponatremia will lead to mental obtundation and seizures. Due to these considerations, intravenous hypotonic solutions should be avoided in the neurointensive care unit.
A self-limited, salt wasting nephropathy can develop after SAH. The process will lead to hyponatremia and volume depletion if not recognized and treated (103). The etiology of this process remains somewhat difficult to identify. In dogs and guinea pigs, the process is mediated through the renal sympathetic nerves; transection of these nerves will prevent salt wasting. This response is species-specific and not true for rats. The human mechanism is unclear (104). A variety of circulating hormones or substances have been proposed to initiate this response; the leading candidate is the B-isoform of atrial natriuretic peptide (ANP) which has been found in some series to be elevated prior to the development of hyponatremia and cerebral vasospasm (105).
Diabetes insipidus (DI) leading to hypernatremia is expected after pituitary surgery and is seen in any process that affects the hypothalamus or pituitary gland. Head trauma leading to a shearing injury of the pituitary stalk is a common cause for delayed DI. The diagnosis is made by the development of hypernatremia in the setting of a nondiuretic-induced hypotonic diuresis. This process must be differentiated from a postoperative diuresis; a normal postoperative diuresis will not spontaneously develop hypernatremia. Correction of the hypernatremia must be done slowly if hypernatremia has been maintained for more than a few hours (102). DI can rarely be seen in SAH patients as well; it is theorized that DI in this situation can be due to vasospasm of the vessels supplying the anterior hypothalamus.
General Therapeutic Considerations
Hypothermia. Hypothermia exerts neuroprotective effects in animal models through various mechanisms. However, its clinical application has been limited by disappointing results in randomized controlled clinical trials. At present, the only evidence-based indications for therapeutic hypothermia are postresuscitation coma—especially after witnessed out-of-hospital ventricular fibrillation arrest—and neonatal hypoxic encephalopathy.
Moderate hypothermia (33°C) was proven to be associated with improved neurologic outcomes in two randomized trials for global cerebral ischemia after cardiac arrest (106,107). Hypothermia must be induced early and maintained for 12 to 24 hours before proceeding to slow rewarming. Sedation and pharmacologic paralysis were instituted to prevent the hypermetabolism that occurs with shivering. A recent trial comparing hypothermia of 33°C versus 36°C demonstrated no difference in primary or secondary outcomes including mortality, functional outcomes in survivors, and adverse effects (108). While the target of temperature control can be debated, the intervention should be considered standard of care.
An initial trial hypothermia in severe TBI showed no difference in outcome between hypothermia and normothermia groups (109). A second trial (n = 232 patients) evaluating earlier induction of hypothermia was terminated because of futility (110); subgroup analysis showed a difference in favor of hypothermia among patients with surgically resectable hematoma (poor outcome 33% versus 69%; p = 0.02), but this was based on a very small number of patients. At this point hypothermia cannot be recommended as a routine treatment for severe TBI.
Hypothermia has been applied to patients with large ischemic stroke and patients with intracerebral hemorrhage (ICH) and associated brain edema in small case series with promising results (111,112). However, multicenter trials are necessary before hypothermia can be recommended for these indications.
Intubation
Medical complications are common after neurologic injury and they worsen outcome (99,113). Aspiration pneumonia is reported with depressed levels of consciousness, and early recognition and treatment are needed. ETI is required for neurologic patients that are unable to maintain airway patency or protect their airway from secretions. ETI portends a poor outcome for patients with ischemic and hemorrhagic strokes (114). The timing of extubation in the neurologic patient is controversial since many patients remain intubated solely for airway protection. The usual practice is to keep patients intubated as long as they remain comatose; yet prolonged intubation in neurologic patients increases the rate of ventilator-acquired pneumonia, increases length of stay, and worsens outcomes. Early extubation based upon the patients’ ability to control secretions is recommended (115). The value of early tracheostomy—by days 5 to 7 after intubation where there is no neurologic improvement—deserves further investigation.
SPECIFIC CONDITIONS AND TREATMENT OPTIONS
Head Trauma
Severe head trauma remains a significant source of morbidity and mortality in the United States accounting for approximately 50,000 deaths/year, and is the leading source of death for people less than 44 years of age (116). Historically, treatment has focused on the management of sustained intracranial hypertension, based largely on retrospective data obtained from the National Traumatic Coma data bank. Survival was greater than 80% in patients in whom the ICP was maintained <20 mmHg, compared to >90% mortality for patients that had uncontrollable sustained intracranial hypertension (117,118). Treatment, therefore, was designed to institute measures to decrease ICP. This could include diuresis, aggressive treatment of hypertension, keeping the patient relatively hypovolemic, and elevation of the head of bed.
The work by the Richmond group in the 1970s to 1980s largely changed the focus on treating head trauma from using measures designed to lower ICP to using measures designed to maintain adequate CPP. A recent study conducted in Bolivia and Ecuador compared ICP guided versus imaging and clinical examination guided therapy and found no significant difference in functional outcome or survival (119). The major criticism of this study is the questionable external validity in developed countries where pre- and posthospital care and resources, including rehabilitation, are plentiful. As per the Brain Trauma Foundation guidelines, ICP guided therapy in TBI is recommended for patients with GCS of 3 to 8 after resuscitation, abnormal CT scan of the head, and with two or more of the following at admission: age over 40, hypotension–systolic blood pressure <90 mmHg, and motor posturing (45).
Treatment thus shifted to include maintaining adequate blood volume and cerebral perfusion as well as treating sustained elevations in ICP. What constitutes an adequate CPP has been debatable and may vary under different conditions; however, the most recent Brain Trauma Foundation guidelines have recommended a CPP range of 50 to 70 mmHg (45).
Several studies have demonstrated an increase in mortality in TBI patients with brain tissue oxygen tension (PbtO2) below 10 to 15 mmHg (120–122). More recently, brain tissue oxygen monitoring has been used in head trauma to guide therapy. Brain tissue oxygenation tension is measured by placement of a small flexible probe through a cranial bolt directly into the brain parenchyma. CBF and PET studies have reported good correlation with low oxygen tensions with low CBF measures and high oxygen extraction ratios (123,124).
A meta-analysis of trials comparing brain tissue oxygen guided therapy versus conventional ICP guided therapy concluded that PbtO2 guided therapy significantly improved neurologic outcome (125). However, the historical controls had a very high mortality. For example, in a study of 53 patients, PbtO2 guided therapy study was associated with decreased mortality compared to historical controls (mortality 25% vs. 44%, p < 0.05) (126); the average mortality rate across trauma centers was similar to the treatment arm rather than the historical control arm of this study. Another study with 139 patients had similar criticisms raised (127). In contrast, a study of 123 patients comparing PbtO2 guided therapy to conventional ICP therapy showed not only no benefit in survival and outcome, but increased overall costs and ventilator-associated days in the treatment arm (128). Yet, these negative results could have been related to selection bias as the PbtO2 monitors were placed at the discretion of the neurosurgeons and the treatment cohort had significantly worse neurologic presentation. The latest Brain Trauma Foundation guidelines from 2007 suggest consideration for the use of PbtO2 monitoring with a level III evidence (45). The more recent Consensus Statement on Multimodality Brain Monitoring from the Neurocritical Care Society endorsed a stronger recommendation for the use of PbtO2 monitoring in patients at risk of cerebral ischemia or hypoxia, such as those with severe TBI, but acknowledged that the quality of evidence to support this recommendation is low (129). The main limitation of PbtO2 monitoring is that it only measures oxygenation in the close proximity of the catheter; location of the catheter should, therefore, be carefully individualized. Aiming for pericontusional tissue is often preferred because this is considered the area at highest risk for secondary injury (130).
A small study (n = 52 patients) evaluating 100% oxygen supplementation for 24 hours following TBI revealed statistically significant improvements in brain glucose, glutamate, lactate, lactate/glucose ratio, and lactate/pyruvate ratio. The ICP was also significantly decreased in patients receiving 100% oxygen. However, 3- and 6-month outcomes were not better than in controls (131). A Cochrane review of hyperbaric oxygen therapy for TBI identified 7 trials including 571 patients found a modest improvement in GCS associated with this treatment, but appropriately concluded that the therapy cannot be currently recommended because the trials were small and unblended, and, consequently, the estimates of benefit are not reliable (132).
Intracranial hypertension is treated through the removal of space-occupying lesions, decreasing cerebral edema or venous engorgement, or expanding the cranial vault. Expanding brain masses—tumors, subdural or epidural hematomas—need to be evacuated as soon as possible to avoid cerebral herniation and damage to important brain structures. Removal of CSF through an external ventricular drain is another means to reduce the intracranial volume. Placement of an external ventricular drain also allows for the direct measurement of ICP.
Hyperventilation is useful for the acute management of intracranial hypertension, as it rapidly decreases CBV through arteriolar vasoconstriction. Chronic hyperventilation is generally avoided due to concerns that prolonged vasoconstriction may worsen cerebral ischemia (133). The effect of hyperventilation on ICP is also self-limited, usually lasting only 3 to 6 hours. Thus, hyperventilation is optimally used for ICP control in the acute setting until some longer-acting strategy can be employed.
Osmotic agents are typically employed to lower ICP. Mannitol (20% solution) given in boluses of 0.25 to 1.0 g/kg lowers ICP through a number of mechanisms. The intravascular osmotic gradient created by mannitol can lead to extracellular fluid being drawn into the intravascular space and removed through an osmotic diuresis. Reductions in hemispheric brain water have been demonstrated in a rat stroke model after the administration of large boluses of mannitol. The effect, however, was small and delayed for several hours (134). More likely, mannitol exerts its effect on ICP by decreasing CBV. According to this theory, the osmotic gradient initiated by an infusion of mannitol causes an influx of extravascular water into the intravascular space. This leads to a decrease in blood viscosity due to a hemodilution of red blood cell mass and fibrinogen. The decrease in ICP can be explained through the use of the Hagen–Poiseuille equation. In this equation, flow is directly related to the fourth power of the radius of the cerebral vessel and inversely related to serum viscosity. If the flow remains constant and viscosity is reduced, then vessel diameter must decrease (39):
where F is the flow; dP is the pressure gradient (CPP); r is the vessel diameter; n is the viscosity; and l is the vessel length. Then, assuming constant CBF and cerebral vessel reactivity, according to this equation, the radius of this vessel must decrease in response to both an increase in CPP and a decrease in viscosity (10). Rosner describes this as a passive vasoconstriction (39).
Hypertonic saline has been increasingly used in the treatment of brain trauma patients and it might be superior to mannitol for this indication (135). Hypertonic saline should also produce less risk of nephrotoxicity. Theoretically, its mechanism of action would be similar to that of mannitol, but hypertonic saline can increase cardiac output and indices less diuresis. Infusion of 30 to 60 mL of 23% sodium chloride is the most effective osmotic therapy to reverse severe surges in ICP and should be preferred in patients with signs of brain herniation (136).
An overall strategy for treating intracranial hypertension is to attempt to maintain the most optimal CPP and the lowest possible ICP. To attain this goal, other maneuvers may need to be used. Sedation and paralysis can be helpful to decrease ICP if chest wall compliance is high or if the patient is exhibiting respiratory dyssynchrony with the ventilator. Since the spinal venous plexus lacks valves, there is a theoretical concern that elevations in peak end expiratory pressures (PEEP) could be transmitted directly to the brain, thus increasing ICP. This is rarely problematic, but can occur if PEEP is maintained greater than 15 to 20 cm H2O under conditions of increased pulmonary and decreased intracranial compliance (137).
Corticosteroids are useful in treating the vasogenic edema from tumors and meningitis. The use of glucocorticoids in head trauma, however, is not recommended as several randomized trials have found no therapeutic benefit (138–140).
Barbiturates are effective in lowering ICP by decreasing cerebral metabolism. Their use is generally reserved for cases of refractory intracranial hypertension. Dosing is often titrated to a burst suppression pattern monitored on the EEG. One randomized trial reported improved mortality (141) with barbiturate use. Barbiturate use in head trauma, however, remains debatable due to the limited quality of life of survivors (142). High-1. Hemicraniectomy with duraplasty can be another alternative in very refractory cases (143). However, bifrontal craniectomy in patients with less severe intracranial hypertension cannot be recommended based on the negative results of the DECRA trial (144).
Seizure Control and Status Epilepticus
Seizures are common after neurologic injury and can worsen outcome by increasing metabolic demands beyond oxygen delivery capabilities. Anticonvulsant prophylaxis may be employed for patients with SAH, or intracerebral hemorrhages that abut or involve the cortical surface of the brain. Anticonvulsants are recommended during the first week after severe head trauma to prevent posttraumatic epilepsy (145). Immediate treatment of seizures should utilize generous dosing of benzodiazepines.
SE is a neurologic emergency associated with significant morbidity and mortality. Traditional definitions of SE that require 30 minutes of sustained seizure activity or nonarousal between sequential seizures have proved impractical to initiating timely treatment. A new operational definition advocates immediate treatment of all seizures that last >5 minutes (146). Protocols have been developed for the sequential application of anticonvulsants (146). Aggressive early initiation of treatment is important because SE becomes more difficult to control as seizures continue. Tachyphylaxis of antiepileptic medications is explained by receptor trafficking resulting in downregulation of GABA receptors and upregulation of NMDA receptors.
Tonic–clonic movements correlating with electrical seizure activity evolve through a progression of clinical stages until a form of electrical mechanical dissociation can occur. Over time, physical movements become progressively more subtle and can be manifested only by slight movements of the lips, fingers, or eyelids. It is important to note that nonconvulsive SE is often underrecognized. A common mistake is to assume seizures have resolved after loading with anticonvulsants and initiating pharmacologic paralysis. This underscores the importance of EEG monitoring to ensure seizures have been adequately treated. Attention to airway management and hemodynamics are important because many of the anticonvulsants will have respiratory and cardiovascular depressant effects.
Refractory SE is defined as SE that has not responded to the usual first- and second-line medications necessitating anesthetic infusions. Super refractory SE is a relatively new terminology to characterize an SE that continues despite 24 hours of anesthetic infusion. Propofol, midazolam, and thiopental or pentobarbital infusions under EEG monitoring are required for treatment. Propofol use has fallen into relative disfavor due to several case reports of deaths during infusions (147); it is not used in children with SE due to concerns over development of a propofol infusion syndrome (148).
Subarachnoid Hemorrhage
SAH from ruptured cerebral aneurysms has a high morbidity and mortality, with 15% of patients dying before reaching medical attention (149).
The care of patients with SAH can be divided into management before and after the ruptured aneurysm is secured. Management prior to aneurysmal treatment is designed to prevent rebleeding, which occurs in up to a quarter of patients with aneurysmal SAH (aSAH) and this risk is the highest within the first 2 days after aSAH. Neurosurgical repair or endovascular coil embolization of the aneurysm is, therefore, instituted as soon as possible.
Most patients will have acute elevations in blood pressure due to the significant pain stress and catecholamine surge that occurs after SAH. The management of blood pressure after SAH is controversial, but most neurosurgeons and neurointensivists will treat acute hypertension in patients with unsecured aneurysms. The rationale for treatment is based on the international cooperative aneurysmal trial (150) and a large Japanese observational study that noted a higher incidence of rebleeding in patients with sustained systolic elevations in blood pressure >160 mmHg (151). Blood pressure is preferentially treated with beta blockers which do not significantly affect CBF and can narrow the pulse pressure; hydralazine and ACE inhibitors have minimal effects on CBF. In general, nitroprusside is avoided due to concerns of cerebral venodilation and increases in ICP. Intravenous nicardipine is the drug of choice if intravenous titration of blood pressure is needed (149).
The use of antifibrinolytic agents (tranexamic acid, aminocaprioc acid) to prevent rebleeding is also controversial. Previously a mainstay of treatment, the use of antifibrinolytic agents was largely abandoned after studies revealed that the benefit from reduction in rebleeding was offset by increases in delayed ischemic complications when the drug was used for many days. However, a more recent randomized controlled trial showed rebleeding rates were significantly decreased without an increase in the rate of ischemic complications when tranexamic acid was started early and continued for no longer than 72 hours (152).
Acute hydrocephalus is common after SAH and requires prompt diversion of CSF through placement of an external ventricular drain or, alternatively, a lumbar drain when there is no obstruction to the CSF flow. Underrecognition of acute hydrocephalus is common and can be very detrimental to patients’ outcomes. Similarly, premature discontinuation of CSF drainage is another common mistake with frequent deleterious consequences.
Once the aneurysm is secured, care focuses on the evaluation and treatment of cerebral vasospasm. Cerebral vasospasm is a pathologic narrowing of the basal cerebral arteries that occurs days after SAH. Pathologically, the cerebral vessels display intimal hyperplasia, collagen remodeling, and a diffuse cellular infiltrate (153). The process takes approximately 4 days to develop and resolves after approximately 2 weeks; it is initiated by some breakdown products of oxyhemoglobin found in the subarachnoid space. The likelihood of developing cerebral vasospasm is directly related to the amount of subarachnoid blood visualized on a 24-hour CT scan (154). Tobacco use and a high Hijdra sum score (>23) are predictive of developing vasospasm (155). The Hijdra sum score allocates 0 to 3 points based on the amount of blood noted in the 10 cisterns and fissures (156); intraventricular hemorrhage also contributes to the risk of vasospasm (157).
Cerebral vessel narrowing can be monitored by the use of TCD ultrasonography. Rising flow velocities often precede the development of neurologic deficits (153). Perfusion scans combined with noninvasive angiograms can be useful when it is unclear if the symptoms are caused by vasospasm. Conventional angiography is the gold standard for diagnosis of large-vessel vasospasm, but may fail to show any major abnormalities in symptomatic patients with vasospasm in more distal vessels.
Symptomatic vasospasm is treated with hemodynamic augmentation therapy, consisting of vasopressors and fluids to maintain isovolemia. Recalcitrant deficits may require interventional angioplasty or intra-arterial infusion of vasodilators—usually calcium channel blockers—to open the narrowed vessels.
Oral nimodipine, a calcium channel blocker, has been shown to improve functional outcomes in aSAH by reducing the risk of delayed ischemia; its routine use is recommended (158). Yet, it is important to make sure that its administration does not compromise the perfusion pressure as this effect will likely negate any benefits and might even increase the risk of infarction in patients with ongoing severe vasospasm.
Intracerebral Hemorrhage
ICH is bleeding that occurs directly into the brain parenchyma; it is classified as primary (or spontaneous) or secondary to underlying lesions. The most common etiology of ICH is hypertension, which weakens and ruptures the small perforating vessels of the basal cerebral arteries. Long-term anticoagulant use is becoming an increasing risk factor for ICH, especially in the elderly (159).
Clinically, ICH presents with severe headaches and focal neurologic deficits usually prompting immediate evaluation in an emergency room. Serial head CT studies have revealed that hematoma expansion occurs in approximate 40% of patients with ICH within the first 20 hours (160). A double-blinded randomized trial showed that the use of recombinant factor VII used within the first 4 hours of ictus decreased hematoma expansion, but did not result in improved functional outcomes and was associated with an increased risk of thromboembolic complications (161).
Acute hypertension is very common after ICH. Aggressive blood pressure control has been controversial because of theoretical concerns that it might cause ischemia around the hematoma. However, PET studies suggest that moderate control of hypertension is safe (162). Furthermore, a large randomized controlled trial comparing target systolic blood pressures of 140 versus 180 mmHg demonstrated a modest functional benefit in the intensive control arm (163). Another study addressing the question of rapid blood pressure control is currently ongoing and scheduled to be completed in July 2016 (ClinicalTrials.gov Identifier: NCT01176565).
A large randomized study evaluating surgical evacuation of supratentorial ICH did not report a benefit over medical management (164). Subgroup analysis did suggest a benefit to early evacuation of superficial hemorrhages. However, a follow-up study failed to show benefit even within this subgroup (165). Minimally invasive surgery with clot lysis using recombinant tissue plasminogen activator (tPA) has been shown to be safe (166). A phase III study is underway and scheduled to complete in September 2019 (ClinicalTrials.gov Identifier: NCT01827046). Corticosteroids have not shown any benefit in the treatment of ICH (167).
Ischemic Stroke
The use of thrombolytics has dramatically changed the management of acute ischemic stroke. The NINDS trial demonstrated improved 3-month outcomes in patients treated within 3 hours of onset with intravenous tPA (168); the treatment window has been expanded to 4.5 hours for select populations (169). Exclusion criteria for the extended window include the use of anticoagulants regardless of INR, age greater than 80, history of stroke, as well as diabetes and NIHSS > 25.
Several recent multicenter trials have shown that intra-arterial thrombectomy, in addition to intravenous alteplase, improves functional outcome in patients with severe acute ischemic stroke caused by a proximal intracranial vessel occlusion (170–172). This is in contrast to some previous endovascular studies which had failed to show benefit from intra-arterial interventions (173–175). The reason for the negative results of earlier studies is thought to be due to utilization of older generation clot retrieval devices and enrollment of patients without proven proximal intracranial vessel occlusion. Endovascular therapy should, therefore, be pursued in patients with disabling deficits from a large artery occlusion who fail to improve with intravenous thrombolysis—or have contraindications for its use—and do not already have a large established infarction on CT scan. For endovascular therapy to be effective, rapid intervention is essential.
Hypertension is common in the setting of an acute stroke and usually resolves within a few hours of ictus. Treatment to slowly decrease blood pressures, although in the current guidelines, has not shown to affect clinical outcome by recent studies (176–178). Hemorrhagic conversion of ischemic infarctions is increased with sustained systolic pressures over 180 mmHg and blood pressure must be below this limit prior to tPA administration (168). However, over-aggressive treatment of blood pressure is commonplace and can worsen the ischemic by compromising collateral flow.
Large hemispheric infarctions, defined as stokes involving over 50% of the middle cerebral artery (MCA) territory, are at risk for the subsequent development of cerebral edema and herniation (179). Close neurologic monitoring is required to identify any signs of deterioration. Treatments designed to lower ICP can be effective, but act as only a temporizing measure since cerebral tissue shifts and not increased ICP is most likely to be the source of neurologic deterioration (180). Hemicraniectomy has been proven effective in reducing mortality and improving functional outcomes in survivors among patients younger than 60 years when completed within 48 hours (181). Benefit is much more modest in older patients for whom decompressive hemicraniectomy can be lifesaving but may not prevent permanent severe disability (182).
SUMMARY
This chapter has attempted to provide an overview of cerebral vascular physiology and cerebral ischemia. A grasp of these principles is vital to understanding the nature of treatments designed to maintain adequate CBF and prevent secondary neurologic injury. Future treatments that focus on the details of critical care and maintaining tissue oxygenation show promise in improving outcome after neurologic injury.
Key Points
- A strong understanding of cerebral physiology is essential to construct a rational approach to the diagnosis and treatment of neurologic emergencies.
- Mechanisms of primary neurologic injury will vary depending upon the nature of the insult and these are often not modifiable. However, most critical neurologic and neurosurgical disease generate a risk secondary injury from cerebral hypoxia and ischemia, which can be ameliorated through optimal management.
- The primary goal of acute neurologic management is to prevent secondary injury.
- Hypoxemia, hypotension, expanding mass lesions, persistent seizures, and intracranial hypertension can all worsen secondary injury. Immediate attention and correction of these problems can have a significant impact on short- and long-term outcomes.
- Cerebral ischemia develops if cerebral oxygen utilization cannot meet the metabolic demand. This mismatch can result from problems with oxygen delivery, increased metabolic demands, or impaired oxygen utilization.
- Hemodynamic management should be adjusted to the specific neurologic diagnosis. Blood pressure goals are entirely different across different acute cerebral diseases and even vary depending on the timing from the acute injury.
- Cardiac stunning and neurogenic pulmonary edema can occur after acute neurologic catastrophes; they are most commonly seen after severe head trauma, SAH, SE, or intracerebral hemorrhage. Neurogenic cardiopulmonary damage is mediated by massive catecholamine release from the central sympathetic nervous system.
- There are multiple methods of invasive and noninvasive monitoring of cerebral function. All modalities have limitations and, consequently, it is best to apply them in combination and avoid interpreting their results in isolation.
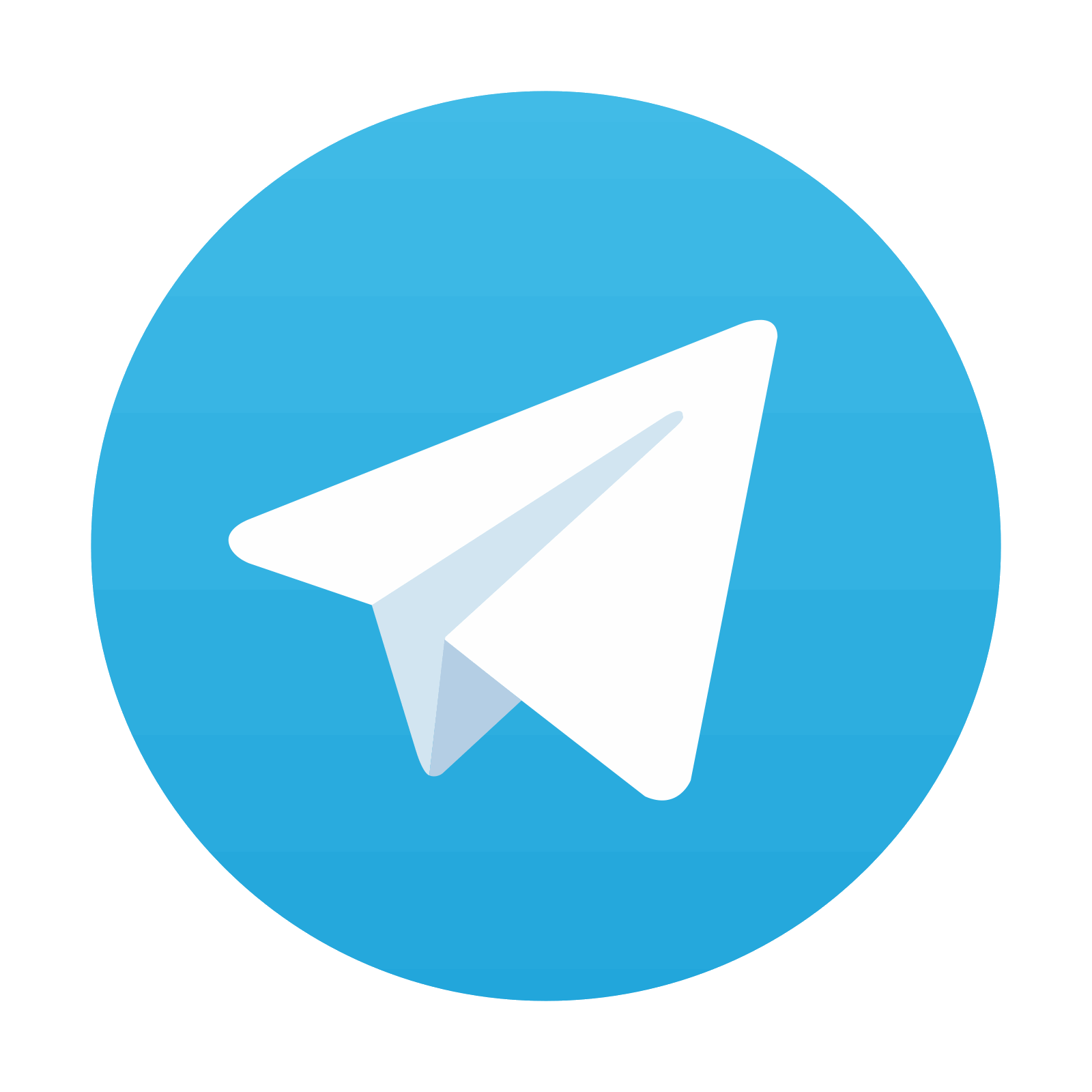
Stay updated, free articles. Join our Telegram channel
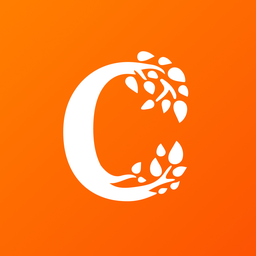
Full access? Get Clinical Tree
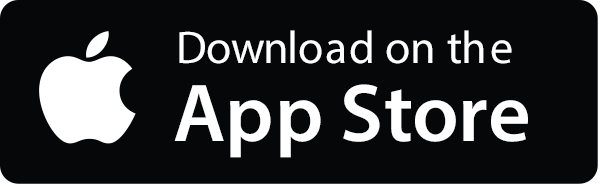
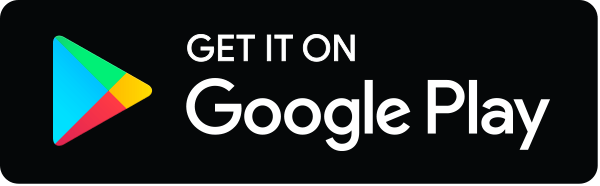