The editors and publisher would like to thank Drs. Lundy Campbell and Michael Gropper for contributing to this chapter in the previous edition of this work. It has served as the foundation for the current chapter.
The central nervous system (CNS) deserves special consideration in the perioperative setting for several reasons. First, many CNS diseases, such as intracranial tumors or aneurysms, are amenable to surgical treatment. Second, many patients presenting for non-neurologic procedures have concurrent CNS diseases such as prior stroke or Parkinson disease. Third, the CNS is metabolically active with little oxygen reserve and is therefore sensitive to ischemia and hypoxia even for very brief periods of time. The latter is especially important in patients with increased vulnerability to complications from cerebrovascular insufficiency or other flow-relevant abnormalities. This chapter discusses the relevant knowledge base and clinical care needed when taking care of patients with CNS diseases in the perioperative setting.
Neuroanatomy
Conceptually, the cranium is divided into supratentorial and infratentorial compartments. The supratentorial compartment contains the cerebral hemispheres and diencephalon (thalamus and hypothalamus), whereas the brainstem and cerebellum make up the infratentorial compartment. In addition, intracranial lesions may be classified as either intra-axial or extra-axial, within or outside the brain parenchyma, respectively. The location of an intracranial lesion has important implications on the anesthetic considerations for that patient and determines the patient’s position during surgery. The location of intra-axial mass lesions is particularly relevant, as some lesions may place eloquent areas such as the language centers and motor cortex of the brain at risk. In this case, functional preservation during surgery becomes critically important.
The arterial blood supply to the brain is through the left and right internal carotid arteries (anterior circulation) and the vertebrobasilar system (posterior circulation). Anastomoses between these vessels form the circle of Willis ( Fig. 30.1 ) and create a collateral blood supply to protect against focal ischemia. However, this ring is not complete in all patients; approximately 20% of the population has an abnormal circulation, implying that the collateralization may not be complete. The clinical significance of an abnormal circle of Willis may depend on the pattern of the abnormality and the coexisting cerebrovascular diseases.

There are 12 pairs of intracranial nerves. It is important to understand the distribution and the sensorimotor and autonomic function of each nerve for the following reasons. First, some neurosurgery procedures may jeopardize specific intracranial nerves during surgery; for example, resection of acoustic neuroma may injure the vestibulocochlear nerve. Second, some of the intracranial nerves are monitored intraoperatively to facilitate timely detection of reversible injury and theoretically prevent permanent deficit. Both somatosensory and motor evoked potentials are frequently monitored intraoperatively. The anatomy of the sensorimotor cortex and pathways is also important for the anesthesia provider to understand.
The anatomic and functional integrity of the blood-brain barrier has important clinical implications. The blood-brain barrier is composed of capillary endothelial cells with tight junctions that prevent free passage of macromolecules or proteins. In contrast, lipid-soluble substances (carbon dioxide, oxygen, anesthetic drugs) cross the blood-brain barrier easily. The blood-brain barrier may be disrupted by acute systemic hypertension, trauma, infection, arterial hypoxemia, severe hypercapnia, tumors, or sustained seizure activity. Osmotic pharmacologic therapy for intracranial hypertension or the need for intraprocedural brain relaxation relies on an intact blood-brain barrier in order to move the free water from the brain parenchyma to the intravascular space.
Neurophysiology
Regulation of Cerebral Blood Flow
Normal cerebral blood flow (CBF) is approximately 50 mL/100 g/min and represents 12% to 15% of total cardiac output. The brain, albeit being 2% of the total body weight, receives a disproportionately large share of cardiac output because of its high metabolic rate and inability to store energy. Some of the important factors or physiologic processes that regulate CBF include (1) cerebral metabolic rate via neurovascular coupling, (2) cerebral perfusion pressure (CPP) via cerebral autoregulation, (3) arterial blood carbon dioxide and oxygen partial pressure (Pa co 2 and Pa o 2 , respectively) via cerebrovascular reactivity, (4) sympathetic nervous activity, (5) cardiac output, and (6) some anesthetic drugs. Different regulatory mechanisms may exert distinctive effects on CBF, which are integrated at the level of cerebral resistance arteries/arterioles to determine the CBF.
Cerebral Metabolic Rate and Neurovascular Coupling
Cerebral metabolic rate of oxygen (CMRO 2 ) is often used as an index of the cerebral metabolic activity. CMRO 2 and CBF are closely related—an increase or decrease in CMRO 2 results in a proportional increase or decrease in CBF. This is named neurovascular coupling or cerebral metabolism-flow coupling. In the perioperative setting, CMRO 2 can be reduced by hypothermia and most intravenous anesthetic drugs, which produce a coupled reduction in CBF in healthy brains. CBF decreases 7% for every 1° C decrease in body temperature below 37° C. In contrast, CMRO 2 and CBF may be dramatically increased by seizure activity.
Cerebral Perfusion Pressure and Cerebral Autoregulation
CPP is the difference between mean arterial pressure (MAP) and intracranial pressure (ICP) or central venous pressure. How CPP affects CBF is determined by cerebral autoregulation, which maintains a stable CBF during a fluctuating CPP as a result of cerebral vasoconstriction or vasodilation in response to an increase or decrease in CPP, respectively. That is, the simultaneous and proportionate changes in CPP and cerebrovascular resistance due to cerebrovascular pressure reactivity lead to a stable CBF. However, as static cerebral autoregulation takes minutes to take effect, a rapid increase or decrease in MAP may cause a brief period of cerebral hyperperfusion or hypoperfusion, respectively.
The cerebral autoregulation curve has three portions: the plateau, the lower limit, and the upper limit ( Fig. 30.2 ). The lower limit is the CPP level below which the CBF decreases linearly with a decreasing CPP. In contrast, the upper limit is the CPP level above which the CBF increases linearly with an increasing CPP. The plateau is the CPP range between the lower and upper limits where CBF remains stable (approximately 50 mL/100 g/min). The frequently quoted limits of autoregulation are a lower limit of 60 mm Hg and an upper limit of 150 mm Hg. However, although these numbers may apply to young and healthy humans, they may not apply in patients with various medical and surgical comorbid conditions. For example, chronic uncontrolled hypertension or sympathetic stimulation shifts the autoregulatory curve to the right. If this is the case, then a higher minimum CPP will be required to maintain an adequate CBF.

Cerebral autoregulation may be impaired or even abolished following traumatic brain injury and intracranial surgery. As a result, CBF becomes pressure passive, implying that it no longer remains stable across the autoregulatory CPP range and instead changes linearly with changes in CPP. Severe hypercapnia, often as a result of hypoventilation, can also impair cerebral autoregulation. Higher inhaled anesthetic concentrations are potent cerebral vasodilators and impair autoregulation. In contrast, intravenous anesthetic drugs do not disrupt this regulatory mechanism. In circumstances when cerebral autoregulation is impaired, the CPP should be carefully controlled because a change in CPP also changes CBF owing to the loss of autoregulatory capability.
Cerebrovascular Pa co 2 and Pa o 2 Reactivity
Both Pa co 2 and Pa o 2 are powerful modulators of CBF and can cause a robust cerebrovascular Pa co 2 – and Pa o 2 -induced reactivity. Changes in Pa co 2 produce corresponding and same directional changes in CBF when Pa co 2 is between 20 and 80 mm Hg ( Fig. 30.3 ). CBF increases or decreases approximately 1 mL/100 g/min or 2% for every 1 mm Hg increase or decrease in Pa co 2 from 40 mm Hg. Such changes in CBF reflect the effect of carbon dioxide–mediated alterations in perivascular pH that leads to cerebral arteriolar dilation or constriction. The Pa co 2 -related change in CBF only lasts for about 6 to 8 hours owing to the compensatory change in bicarbonate (HCO 3 − ) concentration. Both extreme hyperventilation and hypoventilation should be avoided as they can cause cerebral hypoperfusion and hyperperfusion, respectively. Prolonged aggressive hyperventilation following traumatic brain injury is associated with poorer neurologic outcome. In contrast, decreases in Pa o 2 less than a threshold value of about 50 mm Hg result in an exponential increase in CBF (see Fig. 30.3 ), likely a compensatory mechanism to maintain cerebral oxygen delivery (cerebral oxygen delivery = arterial blood oxygen content × CBF).

Effects of Anesthetics on Cerebral Blood Flow
Intravenously administered anesthetic drugs such as propofol and thiopental cause simultaneous reductions of CMRO 2 and CBF. The effect of intravenous anesthetics on CBF is attributed to neurovascular coupling, that is, the decrease in CMRO 2 leads to a corresponding decrease in CBF. The effects of ketamine on cerebrovascular physiology have been variable, which likely reflects different research study conditions. When ketamine is given on its own without control of ventilation, there is an increase in Pa co 2 , CBF, and ICP. However, when ketamine is given in the presence of another sedative or anesthetic drug in patients whose ventilation is controlled, these effects are not noted. Because of this controversy, however, ketamine is usually avoided in patients with known intracranial disease.
Benzodiazepines and opioids decrease CMRO 2 and CBF, analogous to propofol and thiopental, although to a lesser extent. However, associated respiratory depression and increase in Pa co 2 may produce the opposite effect. Opioids should be cautiously given to patients with intracranial disease because of their (1) depressant effects on consciousness, (2) production of miosis, and (3) depression of ventilation with associated increases in ICP from increased Pa co 2 .
α 2 -Agonists (clonidine and dexmedetomidine) are unique sedatives in that they do not cause significant respiratory depression. They decrease arterial blood pressure, CPP, and CBF with minimal effects on ICP. α 2 -Agonists can be used intraoperatively to reduce the dose of other anesthetic drugs and analgesics, or postoperatively as sedatives and to attenuate postoperative hypertension and tachycardia.
In contrast to intravenous anesthetics, volatile anesthetics are potent cerebral vasodilators. When administered during normocapnia at concentrations higher than 0.5 minimal alveolar concentration (MAC), desflurane, sevoflurane, and isoflurane rapidly produce cerebral vasodilation and result in dose-dependent increases in CBF even though CMRO 2 is decreased. Therefore, volatile anesthetics produce divergent changes in CMRO 2 and CBF that are distinct from those of intravenous anesthetics. When used in isolation, nitrous oxide increases CBF and possibly CMRO 2 , however, these effects are attenuated by the coadministration of other anesthetics.
Intracranial Pressure
Determinants of ICP and the Compensation for an Increased ICP
The intracranial compartment normally contains three components: (1) brain matter, (2) cerebrospinal fluid, and (3) blood. Increases in any of these components or the addition of a pathologic lesion (e.g., tumor) can result in an elevated ICP, defined as a sustained increase of ICP higher than 15 mm Hg. Marked increases in ICP can decrease CPP and thereby CBF to the point of causing cerebral ischemia. However, there is a mechanism that restores ICP to normal in the face of an expanding component. This mechanism is accomplished via compensatory reduction of other intracranial components, including the translocation of cerebrospinal fluid from intracranial space to extracranial space. At the moment when this compensatory mechanism is exhausted, ICP starts to increase and cerebral blood vessels are eventually compressed ( Fig. 30.4 ). CBF must be differentiated from cerebral blood volume (CBV) because the former represents flow whereas the latter applies to the intracranial blood volume. These two terms are related but not interchangeable. The treatment of intracranial hypertension is primarily via the reduction of various intracranial components ( Box 30.1 ).

Cerebral Blood Volume Reduction
Decrease Cerebral Blood Flow
Intravenous anesthetic drugs are preferred
Decrease CMRO 2 (propofol, barbiturates)
Employ hyperventilation
Avoid cerebral vasodilators
Avoid extreme hypertension
Increase Venous Outflow
Elevate head
Avoid constriction at the neck
Avoid PEEP and excessive airway pressure
Cerebrospinal Fluid Reduction
External ventricular drain
Lumbar drain
Head elevation (translocation of intracranial cerebrospinal fluid)
Acetazolamide (Diamox)
Cerebral Edema Reduction
Osmotic therapy (mannitol, hypertonic saline)
Furosemide (Lasix)
Prevention of ischemia and secondary edema
Dexamethasone to reduce peritumoral vasogenic edema
Resection of Space-Occupying Lesions
Decompressive Craniectomy
CMRO 2 , Cerebral metabolic rate of oxygen; PEEP, positive end-expiratory pressure.
Effect of Anesthetics on Intracranial Pressure
Most intravenously administered anesthetics reduce CBF, which is associated with a decrease in ICP. The effect of ketamine is controversial and has been discussed previously. These drugs should be considered in patients whose ICP is abnormally increased. However, large doses of propofol or thiopental may decrease systemic blood pressure and CPP. An increased frequency of excitatory peaks on the electroencephalogram (EEG) of patients receiving etomidate, as compared with thiopental, suggests etomidate should be administered with caution to patients with a history of epilepsy, especially considering that seizure increases CMRO 2 , CBF, and ICP as a consequence. Opioids and benzodiazepines reduce ICP through reductions in CMRO 2 and CBF although this benefit will be offset if respiratory depression and an increase in Pa co 2 occur.
As discussed previously, volatile anesthetic drugs are cerebral vasodilators and produce dose-dependent increases in ICP that parallel the increases in CBF and CBV. Hyperventilation to decrease Pa co 2 to less than 35 mm Hg attenuates the tendency for volatile anesthetics to increase ICP. In patients undergoing craniotomy for supratentorial tumors with evidence of a midline shift, neither isoflurane nor desflurane significantly affected lumbar CSF pressure when moderate hypocapnia (Pa co 2 of 30 mm Hg) was maintained. However, these inhaled anesthetics are better avoided in patients with exhausted ICP-compensating mechanisms as evidenced by increased ICP, abnormal mental status, or imaging studies.
Neuromuscular blocking drugs (also see Chapter 11 ) do not usually affect ICP unless they induce release of histamine or hypotension. Histamine can cause cerebral vasodilation leading to an increase in ICP. Succinylcholine may increase ICP through increases in CBF although this is not well documented. Because CBF is coupled to CMRO 2 , a decrease in CMRO 2 leads to a decrease in CBF and thus facilitates the management of intracranial hypertension. Therefore, in patients refractory to initial treatment of intracranial hypertension, a deep level of anesthesia, such as propofol-induced burst suppression or barbiturate coma, can be an alternative option.
Neuroprotection
Many anesthetics have been proposed as neuroprotectants based on their potential to reduce cerebral metabolic rate and excitotoxicity during periods of oxygen deprivation. Many anesthetics, including volatile anesthetics, barbiturates, propofol, and xenon, can provide neuroprotection in animals; convincing human data are lacking. Hypothermia may provide cerebral protection during acute injury. Several animal studies have shown that decreasing body temperature reduces ischemic injury. However, several large prospective, randomized trials of hypothermia in aneurysm surgery and traumatic brain injury have failed to demonstrate such benefit. Yet, cooling patients with return of spontaneous circulation after cardiac arrest improved neurologic outcome in a randomized controlled trial, although a more recent trial contradicted these findings. In contrast, hyperthermia worsens ischemic injury and should be avoided in patients vulnerable to cerebral ischemia.
Neurophysiologic Monitoring
Neurophysiologic monitoring is employed during various neurologic surgeries with increasing frequency because of minimal risk to patients and the potential to reduce intraprocedural neurologic injuries. An understanding of the effects of anesthetics on various monitoring modalities, including the EEG, somatosensory and motor evoked potentials, and intracranial nerve monitoring, is especially critical in neuroanesthesia. The monitoring techniques can be transcranial, direct cortical, or subcortical in approach. Different monitoring modalities often require different anesthetic regimens to preserve the quality of monitoring. Electrocorticography (ECoG) is frequently used during neurologic surgery (also see Chapter 20 ) to identify epileptic foci or activity (afterdischarge) during epilepsy surgery or surgery with intraoperative stimulation mapping. ECoG is sensitive to anesthetic drugs that change the seizure threshold (e.g., benzodiazepines, propofol, and volatile anesthetics).
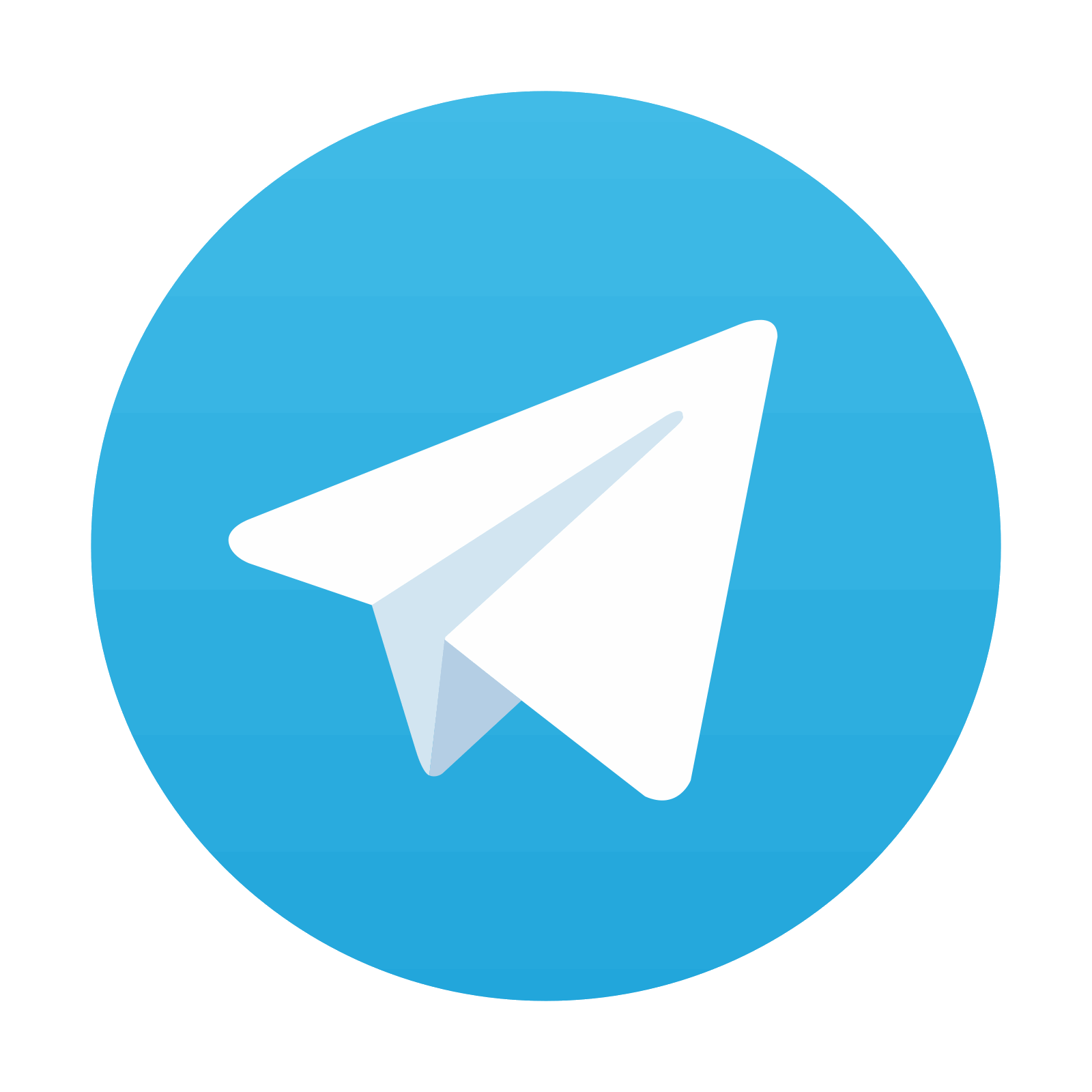
Stay updated, free articles. Join our Telegram channel
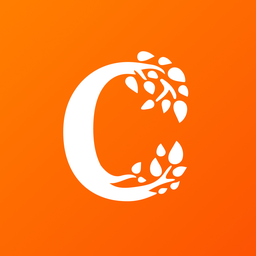
Full access? Get Clinical Tree
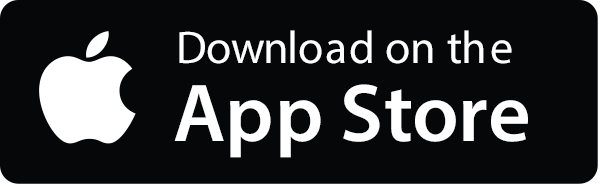
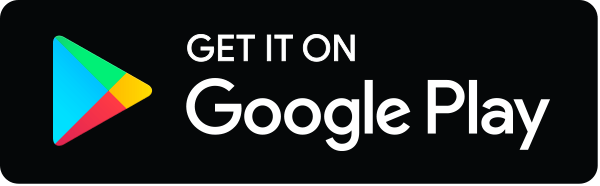