div class=”ChapterContextInformation”>
11. Oxygen Carriers
Keywords
BloodHemorrhageRBCHemoglobinRBC surrogateOxygen carrierIntroduction
In austere battlefield conditions and remote civilian locations, trauma-associated uncontrolled hemorrhage and acute coagulopathy remain one of the leading causes of mortality [1–6]. In such scenarios, transfusion of whole blood and blood components (e.g., RBCs, platelets, and plasma), as per Damage Control Resuscitation (DCR) guidelines, can significantly reduce trauma-associated morbidities and mortalities [7–9]. However, the limited availability and portability, special storage requirements, and high contamination risks of these blood products present severe logistical challenges that preclude facile, ubiquitous pre-hospital application in military and civilian scenarios, for either immediate (e.g., point-of-injury or en route) or prolonged field (e.g., extended, in place) care at the point-of-injury [10–17]. A robust volume of research is currently being dedicated toward resolving these issues and enhancing the availability and applicability of donor-derived blood products in the field [18–21]. In parallel, enabled by transformative advances in the areas of synthetic chemistry, biomaterials, and nanofabrication, an exciting area of research has emerged that focuses on the development and evaluation of semisynthetic or synthetic “bioinspired” surrogates of blood products that can be manufactured at large scale (i.e., sufficient availability); can be sterilized without compromising biofunction, and stored as small volume deliverables over long periods of time across broad ambient temperature ranges and environmental conditions (i.e., easy storage and portability); can be easily reconstituted and administered “on demand” in far-forward scenarios (i.e., pre-hospital applicability); can potentially avoid the need for type matching (universal application with minimal immunogenic risk); can circulate safely upon intravascular administration without systemic risks; and can mimic, leverage, and amplify endogenous mechanisms of blood component function to mitigate the effects of traumatic exsanguinating hemorrhage [22–24]. This field of research has developed in the areas of functionally mimicking blood’s cellular as well as non-cellular components and continues to focus on resolving translational challenges with regard to biocompatibility, safety, pre-hospital availability, and universal applicability.
The research endeavors on preserving and transporting donor-derived blood started during World War I, and blood transfusions became widely available by World War II. Based on this advance, multiple blood banks were established in the USA from the 1950s onward, and blood donation was promoted as a form of civic responsibility. Subsequent development of processes and methodologies for isolation and storage of various blood components has significantly enhanced utilization of whole blood and its components. Currently, transfusions of whole blood as well as various isolated components are clinically approved for application in civilian and battlefield trauma (e.g., in Damage Control Resuscitation), surgical settings (e.g., transplants), chronic and acute anemias, and disease-associated, drug-induced, or congenital bleeding disorders [25–32]. RBC transfusion is clinically significant in efficient mitigation of hemorrhagic shock, as part of the Massive Transfusion Protocol (MTP) in hypoperfused patients with critically limited oxygen delivery [33–37]. It has also been demonstrated that pre-hospital use of RBC transfusion (if available) can significantly improve survival in critically injured subjects [38, 39]. Such transfusions are dependent on donor-derived RBC products (e.g., packed Red Blood Cell or pRBC). However, according to the Red Cross, only ~40% of US population is eligible to donate blood at any given time, and only 10–15% actually donate. In addition, blood-based products have formally limited shelf-life due to accrual of processing and storage-related damage as well as risks of pathogenic contamination. Currently, RBCs have a maximum shelf-life of 42 days, while platelet suspensions have a shelf-life of 5 days, at room temperature [40]. Also, RBCs (and platelets) develop storage lesions over time, which affect their stability, in vivo circulation lifetime, and post-transfusion physiology [41, 42]. Significant research is underway to enhance the shelf-life of blood products by cold storage, freezing, lyophilization, etc. and to develop pathogen reduction technologies like psoralen-based or riboflavin-based UV irradiation, as well as extensive serological testing of donor blood, leukoreduction, and specialized storage protocols [13, 19, 31, 43–48]. Nevertheless, portability of blood products, especially to remote battlefield and civilian locations, especially for pre-hospital point-of-care use, continues to be a major logistical challenge [14, 17, 49].
Such challenges can be potentially addressed by bioinspired engineering of semisynthetic or synthetic surrogates of blood components [22, 50, 51]. In fact, major interest in such synthetic surrogates developed during the HIV crisis of the 1980s due to fear of contaminated blood products, and this research has been ongoing, with several designs and products that have progressed through pre-clinical and clinical evaluations. However, currently no such product is clinically approved by the FDA for human applications in the USA, although certain products have been approved for human use in South Africa and, under special circumstances, dispensed for compassionate use provision in the USA and Europe. Of note, a 2008 meta-analysis of 16 clinical trials of 5 different HBOCs indicated increased risk of both myocardial infarction and death in subjects who received such products [52]. Although this report raised significant concern with regard to the clinical safety and utility of these particular HBOCs, the design of this analysis has been debated and, importantly, this work has directed significant re-emphasis to better understanding the pros and cons of these products at fundamental physiological and mechanistic levels. To this end, the current categorization of such products has shifted from “blood substitutes” to “oxygenation therapeutics” so as to emphasize the important role of such products in scenarios where donor-derived RBCs may not be sufficiently available (e.g., far-forward military setting) as well as for niche scenarios, such as ex vivo perfusion of transplantable organs. In this framework, we will focus on reviewing “hemoglobin-based oxygen carriers” (HBOCs) , comprehensively discussing relevant designs, current state-of-art and novel molecules in development, along with emphasizing criteria for successes and challenges.
Hemoglobin (Hb) Function in RBCs for Oxygen Transport

(a) Multi-scale representation of RBCs and Hemoglobin (Hb), showing a scanning electron micrograph (SEM) image of RBC depicting the biconcave discoid structure, along with sequential schematic of RBC structure, Hb structure, and “Heme” structure; (b) shows a schematic of RBC movement between lung (oxygen loading site) and tissue (oxygen off-loading site), while (c) shows corresponding oxygen equilibrium curve (OEC) characteristics of Hb. (From 2017 Military Supplement: Hemoglobin-based Oxygen Carriers Current State-of-the-Art and Novel Molecules, Anirban Gupta, Shock Injury, Inflammation and Sepsis, Oct 3, 2018, Publish Ahead of Print, Figs. 1–4, with permission of Wolters Kluwer Health, Inc.)
Hb-Based Oxygen Carrier (HBOC) Systems
HBOCs are semisynthetic systems that utilize biologically generated Hb as the oxygen-carrying component and are formulated either as chemically modified cell-free suspensions or conjugated and cross-linked with polymers along with protective enzymes or encapsulated within microparticulate or nanoparticulate vehicles [51, 57]. The Hb used in these systems is usually derived from outdated human or bovine RBCs or from recombinant sources [57–63]. In the case of outdated human or bovine RBCs, the Hb is isolated via cell lysis, purified by sterile filtration and chromatographic techniques, and sterilized (e.g., by low heat) [64]. Using cell-free Hb presents the advantage of minimum antigenicity and improved oxygen diffusivity, due to the lack of interference by cell membrane. In fact, reported in the early twentieth century, suspension of cell-free Hb in lactated Ringer’s solution was used to intravenously treat 15 patients; however, a large number of them developed renal toxicity and cardiovascular complications [64]. Similar results were also found in the 1950s when US Navy treated several patients with cell-free Hb [65]. Cell-free Hb was also found to have a very short circulatory residence time because the Hb tetramer rapidly dissociates into dimeric and monomeric forms that can bind non-specifically to plasma proteins or are captured by scavenging proteins devoted to this purpose (haptoglobin, hemopexin) and thus undergo rapid clearance by the reticulo-endothelial system (RES) into spleen and liver, as well as renal clearance into kidneys, leading to Hb-induced toxicities in these organs [66, 67]. Additionally, cell-free Hb and its dissociated derivatives can also extravasate into the subendothelial domain of the circulatory system and rapidly sequester nitric oxide (NO), resulting in its conversion into nitrate (dioxygenation reaction) and of oxy-Hb to MetHb [68]. NO is an essential endogenous vasodilator (e.g., endothelial-derived relaxant factor) and therefore such NO scavenging results in vasoconstriction and cardiovascular complications, paradoxically, thereby diminishing tissue O2 delivery. Furthermore, 2,3-DPG absence in plasma (as well as lack of Bohr and Haldane-based OEC “shifting,” which is enabled in “intact” RBCs) leads to unnaturally high cell-free Hb oxygen affinity, limiting O2 off-loading across physiologic O2 gradients—further diminishing tissue O2 delivery. Cell-free Hb can also change blood osmolarity, leading to alteration of blood volumes and associated side effects. Altogether, for these reasons, cell-free human Hb appears problematic for in vivo oxygen-carrying applications. Instead of human Hb, studies have also been conducted with bovine Hb, but this also presents similar issues of stability, extravasation, NO scavenging, and renal clearance and toxicity. Historically, an innovative approach to address some of these issues was by development of “designer” recombinant Hb (e.g., in E. coli) where targeted mutations decrease the likelihood of tetramer dissociation, optimize heme redox behavior, and modulate propensity for NO consumption, but an optimal combination of mutations that results in free-Hb performance similar to that observed for intraerythrocytic Hb remains elusive [69–71]. Recombinant technologies are also prohibitively expensive, in comparison to human or bovine sourcing for Hb. Therefore, a substantial volume of research has been directed to achieve in vivo stabilization and performance optimization via chemical modification of purified Hb utilizing techniques (alone or in combination) such as cross-linking, polymerization, and macromeric surface conjugations. The goals of these modifications are to reduce Hb dissociation, extravasation, and renal clearance, while maintaining reasonable circulation lifetime and O2-transport capacities.
Chemically Modified HBOCs
Hb tetramers can be cross-linked both intra- and intermolecularly. For example, intramolecular cross-linking in human Hb formed between its two α-subunits using acylation with bis-(3,5 dibromosalicyl)-fumarate (also known as Diaspirin) led to a product called HemAssist from Baxter, USA [57, 72, 73]. This product showed an increase in circulation residence time up to 12 h compared to <6 h for unmodified Hb, but in human trials, cross-linked Hb unfortunately led to a 72% increase in mortality compared to saline, and clinical trials were discontinued [74]. An analogous approach to cross-link the α-subunits of recombinant Hb using Glycine led to a product called Optro from Somatogen, USA, but this also resulted in increased risks of cardiac arrest and mortality [75–77]. Instead of site-specific intramolecular cross-linking only, polymerized Hb has also been created from using bifunctional cross-linking reagents like glutaraldehyde-based cross-linking of bovine Hb (e.g., Hemopure originally from Biopure, USA, now HbO2 Therapeutics, USA) or human Hb (e.g., PolyHeme from Northfield Labs, USA) and o-raffinose-based cross-linking of human Hb (e.g., the product HemoLink from Hemosol, Canada) [78, 79]. Such polymeric cross-linking creates higher-molecular-weight cell-free Hb that retains “simple” oxygen-binding properties (physiologic OEC shifting is not retained) while minimizing dissociation and rapid clearance observed for unmodified Hb tetramers, when free in plasma. One challenge in these approaches is to precisely control polymer molecular weight and geometry, and rigorous purification steps are necessary to ensure product quality. PolyHeme was reported to progress into Phase III clinical trials in the USA in treating trauma-associated blood loss and showed a decreased need of natural blood transfusions [77]. Clinical trials with HemoPure also showed a reduced need of additional blood transfusions in cardiac surgery [80]. HemoPure has received clinical approval in South Africa and Russia for acutely anemic human patients and is occasionally used on a compassionate basis in the USA. An analogous product from the same company (HbO2 Therapeutics, USA) called Oxyglobin is currently approved in the USA for veterinary use, but has not gained widespread acceptance. HemoLink also advanced to Phase III clinical trials but was discontinued in 2003 when patients receiving treatment experienced adverse cardiac events. In fact, as noted in the meta-analysis which led to FDA stopping clinical trials for this HBOC class, all of these products in their clinical studies have shown various degrees of transient hypertension, organ damage through microvascular constriction and dysfunction, gastro-intestinal distress, nephrotoxicity, neurotoxicity, and increased mortality [52, 80–82].

Representative approaches and design schematics for HBOCs based on chemical modification (cross-linking, surface modification, polymerization, etc.) of Hb that have undergone significant pre-clinical and clinical evaluation. (From 2017 Military Supplement: Hemoglobin-based Oxygen Carriers Current State-of-the-Art and Novel Molecules, Anirban Gupta, Shock Injury, Inflammation and Sepsis, Oct 3, 2018, Publish Ahead of Print, Figs. 1–4, with permission of Wolters Kluwer Health, Inc.)
Encapsulated HBOC Systems
During the past two decades, particulate drug delivery platform technologies (microparticles and nanoparticles) have revolutionized the packaging and delivery of pharmaceutical compounds, by encapsulating active compounds/biologics to protect them from plasma-induced effects, increase their circulation time, and allow sustained availability to cells, tissues, and organs. This design concept has also been adapted to create HBOCs that encapsulate Hb within suitable particulate vehicles. In fact, the pioneering concept and demonstration of “bio-artificial cells” was presented as early as the 1950s and 1960s by Chang and colleagues, by encapsulating Hb as well as other proteins and enzymes within polymeric membrane-based microvesicles. The membrane material originally used was collodion (cellulose nitrate) and later changed to biodegradable polyethylene glycol-polylactide (PEG-PLA) [98, 99]. These Hb-loaded microvesicles, aptly termed “hemoglobin corpuscles,” showed oxygen equilibrium curves similar to RBCs and also allowed coencapsulation and activity of RBC-relevant enzymes like 2,3-diphosphoglycerate (2,3-DPG), carbonic anhydrase, and CAT [100–102]. However, in these systems, a major challenge was posed by the rapid macrophagic uptake and clearance of these micrometer-sized vesicles from circulation, resulting in suboptimal circulation residence time for in vivo use. Reducing the diameter to ~1 micron only marginally improved the circulation lifetime, and a significant research effort has been directed toward further improving the vascular residence time by modifying the surface of the vesicles with lipids and polysaccharides. In another similar design approach, Djordjevich et al. reported on encapsulation of Hb in micron and submicron size lipid vesicles (liposome-encapsulated Hb or LEH), with membrane made of phospholipids and cholesterol [103–105]. A number of variations of this design have followed, e.g., “neohemocytes,” “TRM-645 Neo Red Cells,” etc., where the primary focus has been to maintain uniform Hb-encapsulation levels and uniform size distribution of the vesicles, minimize vesicle destabilization or fusion over time, and enhance storage stability of the vesicles while maintaining the RBC-analogous oxygen transport properties of the encapsulated Hb [106–108]. During the 1990s, the “Stealth Liposome” technology was clinically established, where lipid nanovesicles (100–200 nm in diameter) were surface-functionalized with polyethylene glycol (PEG) to enhance storage stability, reduce opsonization, and prevent rapid macrophagic uptake, and this significantly enhanced the circulation residence time [109, 110]. Consequently, this technology was adapted to form Hb-encapsulated PEG-ylated liposomal vesicles (HbV) [111–113]. 1,2-Dioctadecadienoyl-sn-glycero-3-phosphatidylcholine (DODPC) was used as the major membrane phospholipid for HbV preparation, such that γ-irradiation-induced radiolysis of water molecules in the vesicles generated hydroxy (-OH) radicals that promoted intermolecular polymerization of dienoyl groups to produce highly stable liposomes that could withstand freeze-thawing, freeze-drying, and rehydration processes . The HbV design has shown substantial improvement of circulation lifetime (~60 h in some animal models), and several refinements of this design have been recently reported [114–118]. The oxygen transport ability of these HbV systems was found to be similar to natural RBCs, with comparable oxygen saturation and release kinetics. Also, the liposomal encapsulation of Hb attenuated its NO scavenging effect and thereby appears to reduce the associated negative effects on vasculature. Hb encapsulation in liposomal vesicles also prevented glomerular clearance of Hb (since liposomes are too big for renal clearance) and therefore reduced nephrotoxicity. The current optimized HbV product contains about 30,000 Hb molecules encapsulated within one PEG-ylated liposomal vesicle of ~250 nm in diameter. In comparison, a natural RBC is ~7 μ in diameter and ~2 μ in thickness, containing about 250 million Hb molecules. HbVs have undergone extensive pre-clinical evaluation in suitable animal models for potential use as an RBC surrogate in transfusion and resuscitative mitigation of massive hemorrhagic shock and hemodilution incidents, and oxygenation of ischemic as well as transplanted tissues and organs. Although these studies have shown promise of HbVs as RBC surrogate oxygen carrier, these systems still present issues of broad size distribution of the vesicles, variation in Hb-encapsulation efficiencies, as well as variable pharmacokinetics and complement-mediated immune response in vivo. Further research is currently directed toward resolving these issues for potential clinical translation of HbV designs as well as other analogous designs of liposome-encapsulated hemoglobin (LEH) systems as RBC surrogates (114–118). Interestingly, instead of encapsulating Hb, others have attempted to encapsulate oxygen (O2) directly within phospholipid microvesicles (2–4 μm in diameter) to deliver O2 to deoxygenated RBCs in circulation [119, 120]. Although these oxygen-loaded microbubbles were found to be stable for a few weeks in storage with only small extent of oxygen loss, in vivo they were found to have a very short circulation lifetime (<1 h). Therefore, treatment with these systems would require multiple dosing, which may prompt negative effects of dysregulated oxidative stress and associated toxicity and immune response. Therefore, long-term safety profile of such technologies needs to be rigorously evaluated.

Representative approaches and design schematics for HBOCs based on encapsulation of Hb in microparticle and nanoparticle systems that have undergone significant pre-clinical evaluation and hold clinical promise. (From 2017 Military Supplement: Hemoglobin-based Oxygen Carriers Current State-of-the-Art and Novel Molecules, Anirban Gupta, Shock Injury, Inflammation and Sepsis, Oct 3, 2018, Publish Ahead of Print, Figs. 1–4, with permission of Wolters Kluwer Health, Inc.)
Novel Molecules and Designs Incorporating Hb as O2 Carrier
The focus of this section is to not distinguish between “chemically modified” and “encapsulated” Hb systems, but rather describe and review emerging novel designs and technologies that incorporate Hb for oxygen transport purposes. In one interesting approach, instead of Hb, PEG-ylation was carried out on bovine carboxyhemoglobin (CO-Hb), and the resultant PEG-CO-Hb system has been evaluated for oxygen transport (and CO transport) properties [128–130]. The rationale behind this design is that endogenous CO produced from (hypoxia-enhanced) heme-oxygenase activity is reported to render cytoprotective and homeostatic effects, such as inhibition of apoptosis and inflammation and reduction of oxidative stress and vasodilatory activity [131]. The PEG-CO-Hb product (Sanguinate, Prolong Pharmaceuticals, South Plainfield, New Jersey, USA) has undergone pre-clinical evaluation in small animal models, and is now in Phase I/II clinical trials for sickle cell anemia, thrombotic thrombocytopenic purpura (TTP), and ischemia after subarachnoid hemorrhage, with promising safety profile and oxygenation parameters . In another approach, core-shell cluster structures were formed by conjugating human serum albumin (HSA) on Hb using Hb surface lysines conjugated to HSA cysteine-34 using α-succinimidyl-ε-maleimide cross-linker [132]. These Hb-HSA clusters reported lower risk of rapid clearance and extravasation and thus improve circulation residence time. Further modification of these Hb-HSA core-shell nanoclusters was recently reported in which anti-oxidant enzymes and platinum nanoparticles were embedded in HSA pockets for Hb protection [133]. Thus far, this nanocluster design has been evaluated only in vitro, for oxygen-binding capacity, redox properties, and stability, with promising results. However, rigorous in vivo pharmacokinetics, toxicology, biodistribution, and oxygenation studies, along with demonstrating batch-to-batch compositional and functional consistency, are needed to establish in vivo utility. In another approach, Hb has been loaded in microparticles by coprecipitation with calcium carbonate (CaCO3), followed by glutaraldehyde cross-linking and CaCO3 dissolution, resulting in Hb payload density approaching that of RBCs [134]. However, although these Hb microparticles demonstrate oxygen equilibrium kinetics similar to free Hb (affinity too high for O2 release under physiologic conditions), the circulation lifetime is significantly extended, compared to free Hb. Analogous Hb microparticles carrying about 80% Hb content compared to RBCs have been reported where Hb and MnCO3 were coprecipitated, immediately followed by human serum albumin addition for encapsulation and stabilization of the particles [135]. These particles have shown reduced risks of NO scavenging and associated effect on vasoconstriction. In yet another recent approach, Hb was covalently conjugated directly to the hydrophobic or hydrophilic domain of block-copolymers, and the resultant conjugates were self-assembled to form Hb-loaded micelles [136, 137]. In another interesting design, MnCO3 nanoparticles were used as templates to deposit layer-by-layer (L-B-L) assemblies of Hb and dialdehyde heparin (DHP), followed by cross-linking to stabilize the layers and selective dissolution of the template core [138]. A similar approach was also used to form L-B-L-coated nanotubes where alternate layers of Hb, DHP, and the enzyme CAT were deposited, to create systems for potential application in treating oxidative stress [139]. These complex nanostructures have been characterized in vitro for their morphology, stability, cytotoxicity, and in some cases biofunctionality, but pre-clinical evaluation for oxygen carrying efficacy in vivo is not yet reported. Another recent exciting development in the area of novel HBOC molecules is the utilization of large-molecular-weight extracellular Hb isolated from marine invertebrates like polychaete annelid (e.g., the product HEMOXYCarrier from Hemarina, France) [140]. Pre-clinical studies with this unique Hb molecule have shown reduced microvascular vasoconstriction and no significant impact on mean arterial blood pressure, compared to other HBOCs that utilize bovine or human Hb [139]. Further investigation of this system is currently ongoing to evaluate its potential as a clinical oxygen carrier therapeutic system.

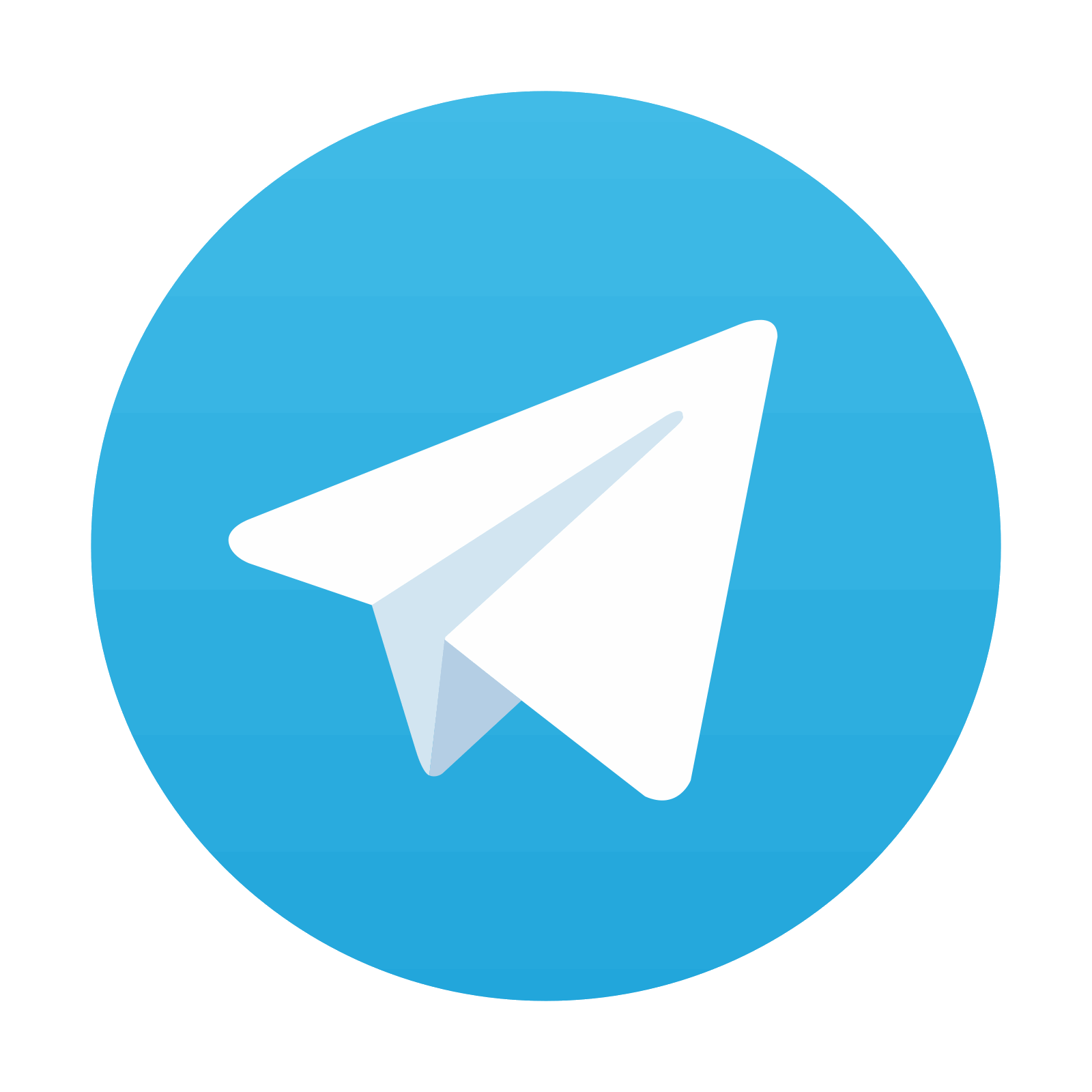
Stay updated, free articles. Join our Telegram channel
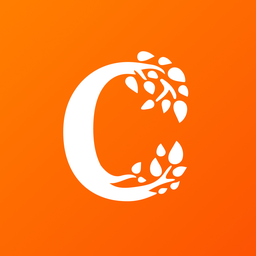
Full access? Get Clinical Tree
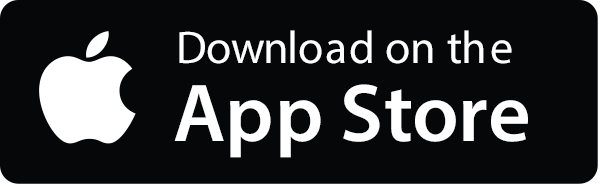
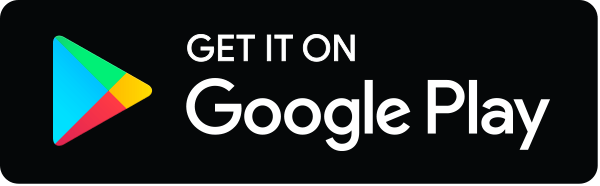