FIGURE 15.1 Internal structure of cardiomyocytes. The electron micrograph shows sarcomeres (SMs) and mitochondria (M), the dominant intracellular organelles. Sarcomeres form rods, which are surrounded by a web of sarcoplasmic reticulum (SR). Sarcomeres and mitochondria are oriented in parallel.
The AP in ventricular cardiac myocytes has a markedly different time course. As an AP passes from the conduction system to the ventricular cardiac myocytes, the voltage-gated Na+ channels provide the positive inward current that depolarizes the ventricular myocyte. The entry of Na+ is rapid, as can be seen from the fast upstroke of the AP, which has been named phase 0, and is due in part to the kinetic characteristics of the voltage-gated Na+ channel, which shows rapid activation and rapid inactivation (Fig. 15.4). The membrane potential moves toward the Nernst potential for Na+, ENa+. Phase 1 describes the notch in the AP that is seen at the initial reversal of the depolarization and is due to Na+ channel inactivation and the transient outward flow of K+ and inward flow of Cl–. However, at this time, complete repolarization is delayed due to the opening of L-type voltage-gated Ca2+ channels, allowing the influx of Ca+ and resulting in a plateau of the AP, known as phase 2. At the plateau, the membrane potential is held near 0 mV for about 100 milliseconds, which leads to the activation of an outward K+ current. Phase 3 describes the termination of the AP and the repolarization of the cell with the outflow of K+ ions due to the opening of K+ channels contributing to the delayed rectifier K+ current. At phase 4, the cell has returned to its resting membrane potential, reestablishing its ion gradients with the activity of the Na+-K+-ATPase pump and the Na+–Ca2+ exchanger. Ionic channels and currents that work in concert to accomplish the cardiac AP and its cyclical automaticity have highly complex organizational flows and structures that we are now beginning to understand. There are additional channels and mechanisms in the process of further investigation.

FIGURE 15.2 A schematic representing a cardiac myocyte demonstrating a few of the important Ca2+ regulatory sites. TnC represents troponin C and RyR represents the ryanodine receptor.

FIGURE 15.3 The surface electrocardiogram at the top of the figure and the action potential profiles throughout the heart and their temporal relationships to each other. SA, sinoatrial; AV, atrioventricular. (From Lynch C. Cellular electrophysiology of the heart. In: Clinical Cardiac Electrophysiology: Perioperative Considerations. Philadelphia, PA: JB Lippincott; 1994:1; with permission.)

FIGURE 15.4 The ventricular cardiac myocyte action potential (AP). The numbers along the AP indicate the phases of the AP. The lower panel schematically represents the relative quantity and temporal relationship of the ionic movements involved in the AP.
Given the importance of Na+ and K+ ion flow in the AP, any inborn or acquired defects in these channels may cause grave pathology. Over the past 20 years, multiple genes encoding for aberrant Na+ and K+ channels have been found to be responsible for inherited arrhythmias such as Brugada syndrome and long and short QT syndrome (4). These ion channel abnormalities, also known as cardiac channelopathies, can cause life-threatening arrhythmias such as ventricular tachycardia or fibrillation.
AUTONOMIC CONTROL OF THE CARDIAC ELECTRICAL ACTIVITY
The autonomic nervous system plays a major role in controlling the initiation of the heart beat and the rate of pacemaker firing. Both parasympathetic and sympathetic nervous inputs converge on the SA and AV nodal cells, exerting opposite influences on heart rate (5).
Parasympathetic Nervous System
The parasympathetic nervous system contributes nerve fibers from its cranial outflow through the cervical ganglia where preganglionic fibers course down to the cardiac plexus, and from there send postganglionic unmyelinated axons that impinge on the SA and AV nodal cells. The cardiac plexus is divided into a superficial and deep plexus; the superficial plexus is found at the base of the heart at the arch of the aorta, while the deep plexus is found on the anterior aspect of the trachea near its bifurcation. The parasympathetic fibers, carried by the vagus nerve, are cholinergic and release acetylcholine (ACh) when activated. ACh has three principal actions that result in the slowing of heart rate and a decrease in contractility: (a) activation of M2 muscarinic receptors in the SA and AV nodal cells, which (b) triggers a reduced spontaneous diastolic depolarization rate, which (c) reduces the slope of phase 0 of the AP, and thus results in a slower heart rate (5).
Sympathetic Nervous System
Sympathetic nervous input to the heart derives from preganglionic neurons in the upper four or five thoracic spinal segments. Axons pass to postganglionic neurons in thoracic and cervical ganglia. The cervical ganglia supply the superior, middle, and inferior cardiac nerves to the cardiac plexus, where they meet the thoracic cardiac nerves from the thoracic ganglia. Sympathetic nervous outflow then supplies the pacemaker cells in the SA and AV nodes, the conduction system, and both the atrial and ventricular myocytes. Norepinephrine, the major adrenergic synaptic mediator in the heart, activates specialized cardiac adrenergic receptors, the most important of which are the β1, β2 and α1 receptors. Activation of β1 and β2 receptors leads to cardiac acceleration and increased contractility. Activation of the α1 receptor also increases contractility. Table 15.1 illustrates the effects of adrenergic receptor activation.
TABLE 15.1 Main Effects of Receptor Activation of Adrenoreceptors | |
![]() |
β-Adrenergic stimulation additionally leads to increased activation of If and accelerates diastolic depolarization, thus increasing the slope of phase 0 of the AP, so that a threshold is reached earlier. IK activation and repolarization are thus faster, with the net result more frequent firing of the AP and a faster heart rate (5). Recent work has focused on the primacy of Ca2+ in regulating the pacemaker function of the SA node (6,7). When mechanoreceptors in the left and right atria sense increased venous return and resultant atrial stretch, an increase in sympathetic-mediated heart rate, known as the Bainbridge reflex, induces tachycardia in order to increase cardiac output (CO) and decrease intracardiac blood volume.
If both parasympathetic and sympathetic inputs to the heart are totally blocked, the heart rate actually increases due to the overriding parasympathetic inhibition seen in most individuals (5).
CONTRACTION–RELAXATION CYCLE
Initiating Events
The spontaneous and rhythmic electrical activity of the pacemaker cells must be transformed into regular and synchronized contraction and relaxation by the atrial and ventricular myocytes through a process described as excitation–contraction coupling. The electrical signal is uniformly passed through gap junctions from cardiac myocyte to cardiac myocyte, producing the AP. The unique characteristic of the AP essential for the initiation of the contractile process is the plateau phase (8). The plateau phase (Fig. 15.5) is due to the prolonged opening of the L-type Ca2+ channel, which provides an inward positive current of Ca2+, thus maintaining the depolarization for a prolonged period. The entry of Ca2+ through the L-type channel initiates the sequence of events leading to contraction.

FIGURE 15.5 Proposed model of sarcoplasmic reticulum (SR) Ca2+ transport as a cardiac pacemaker. After a normal Ca2+ release, the SR Ca2+ uptake refills the SR with Ca2+ and triggers, via the ryanodine receptor, a local SR Ca2+ release, which triggers a Na+–Ca2+ exchange current leading to the depolarization of the sinoatrial nodal cell. SL, sarcolemma; RyR, ryanodine receptor; SR, sarcoplasmic reticulum; PLB, phospholamban. (From Bers DM. The beat goes on. Circ Res. 2006;99:921; with permission.)

FIGURE 15.6 The sarcomere, the minimal unit of contraction in the cardiac myocyte. The A band demonstrates the overlap of thick and thin filaments. The I band represents the thin filaments anchored to the Z line.
Role of Calcium
The Ca2+ pump in the SR, known as the SERCA (sarcoendoplasmic reticulum calcium pump), transports Ca2+ from the cytosol back into the SR. Together with the Na+–Ca2+ exchanger sarcolemmal protein, the SERCA sequesters Ca2+ in the SR in anticipation of the excitation–contraction–relaxation process.
Molecular Interactions
Ca2+ triggers the contractile process by interacting with the Ca2+-binding protein, troponin C, which is an integral part of the sarcomere. The sarcomere is the smallest contractile unit and is defined from Z line to Z line (Fig. 15.6). The myofibrillar structure is made up of interacting filaments, termed thick and thin filaments. Thin filaments are polymers of actin monomers that are anchored to the Z line. The thick filament consists of myosin, a large protein made up of six subunits (Fig. 15.7). The thin filament consists of individual actin molecules that combine to form long polymer chains in a double helical array. Interposed along the actin double helix are complexes of tropomyosin (Tm) and troponin (Tn) (Fig. 15.8). Tropomyosin is a linear molecule that lies in the groove of the actin double helix. Tn is found at the amino terminal end of the Tm molecule. Tn consists of a complex of three protein components: TnT, TnI, and TnC. Each of these components has a unique function essential for contractility. TnT contains the binding site for tropomyosin and allows the Tn complex to be bound to Tm. TnI is an inhibitory subunit. TnC is a molecular switch that undergoes Ca2+-mediated conformational change and activates the actin–myosin interaction (9,10).

FIGURE 15.7 A schematic of the myosin molecule. A two-headed molecule with a flexible neck and an α-helical tail. The head contains a binding site for adenosine triphosphate. The head and neck have attached essential and regulatory light chains that are referred to in the text.

FIGURE 15.8 Schematic representation of protein interactions comprising the contractile apparatus in the cardiac myocyte. (From Ruegg JC. Cardiac contractility: how calcium activates the myofilaments. Naturwissenschaften. 1998;85:575; with permission.)
Cardiac Contraction Cycle
At the molecular level, contraction and force generation occur because of the interaction of the myosin head with actin (11). Two processes control contractility in the cardiac myocyte: the length of the sarcomere, and the intrinsic contractility of the contractile elements (12).
Positive peak dP/dt, the time differential of ventricular pressure, has been employed as a simple index of contractile function. While dP/dt is a simple concept, it is technically challenging to obtain, and is clearly dependent on preload, afterload, and heart rate. A further measure of contractility is the end-systolic pressure–volume relationship (ESPVR) (13). The ESPVR is derived from the pressure–volume loop, an illustration of ventricular volumes plotted against ventricular pressures over the events of a single cardiac cycle (Fig. 15.9). As can be seen in Figure 15.10, pressure volume loops may be generated at different ventricular volumes, and the slope of the line connecting the point of end systole on a family of loops gives a measure of contractility. However, even this measurement of contractility has been shown to yield inconsistent results (11).
Currently, one invasive measure of contractility that appears most consistent is the preload recruitable stroke work (PRSW) relationship. First proposed by Sarnoff and Berglund in 1954 (14), PRSW has been proven to have a linear relationship to the end-diastolic volume (EDV) (15). This index measures contractility despite changes in preload and afterload. The PRSW is obtained from the integrals of a family of pressure–volume loops, the measure of stroke work (SW), at varying EDVs (16–19) (Fig. 15.11).
In clinical practice, pressure–volume loops are not routinely performed due to their time-consuming nature, need for specialized catheters, and manipulation of the different loading conditions of the heart. With that caveat, pressure–volume loops provide a graphic representation of the active and passive properties of the heart as a pump. The shape and position of the pressure–volume loops have been of great value in characterizing the clinical picture. As shown in Figures 15.12 and 15.13, a particular pathologic state can be identified by the pressure–volume loop (20). The pressure–volume loop for patients with dilated cardiomyopathy and restrictive cardiomyopathy are markedly shifted to the right, while in hypertrophic cardiomyopathy, they are shifted to the left compared to normal individuals. Acute coronary ischemia also markedly alters the pressure–volume loops.

FIGURE 15.9 The pressure–volume loop. The y axis represents the pressure in the left ventricle, while the x axis represents the volume in the ventricle. Systole begins with the closure of the mitral valve and initiation of isovolumic contraction at time A. The aortic valve opens at time B for ventricular ejection. Systole occurs from time A to time C, aortic valve closure. Following time C, there is isovolumic relaxation and mitral valve opening at time D, followed by ventricular filling until time A. Diastole occurs from time C to time A. (From Lewis AM. Cardiovascular physiology: flow-volume loops. In: Griffin B, ed. The Cleveland Clinic Cardiology Board Review. 2nd ed. Philadelphia, PA: Lippincott Williams & Wilkins; 2013:37; with permission.)
At the systemic level, the pressure–volume loop can be integrated over time by the eponymous Wiggers diagram of the cardiac cycle, as seen in Figure 15.14. The Wiggers diagram depicts the temporal relationship between cardiac flow and pressure changes, electrical activity, and auscultated heart sounds.

FIGURE 15.10 The pressure–volume loops in different ventricular volumes. The line connecting pressure–volume loops at end systole is a straight line called the end-systolic pressure–volume relationship. (From Sagawa K. The end-systolic pressure-volume relation of the ventricle: definition, modification, and clinical use. Circulation. 1981;63:1223; with permission.)
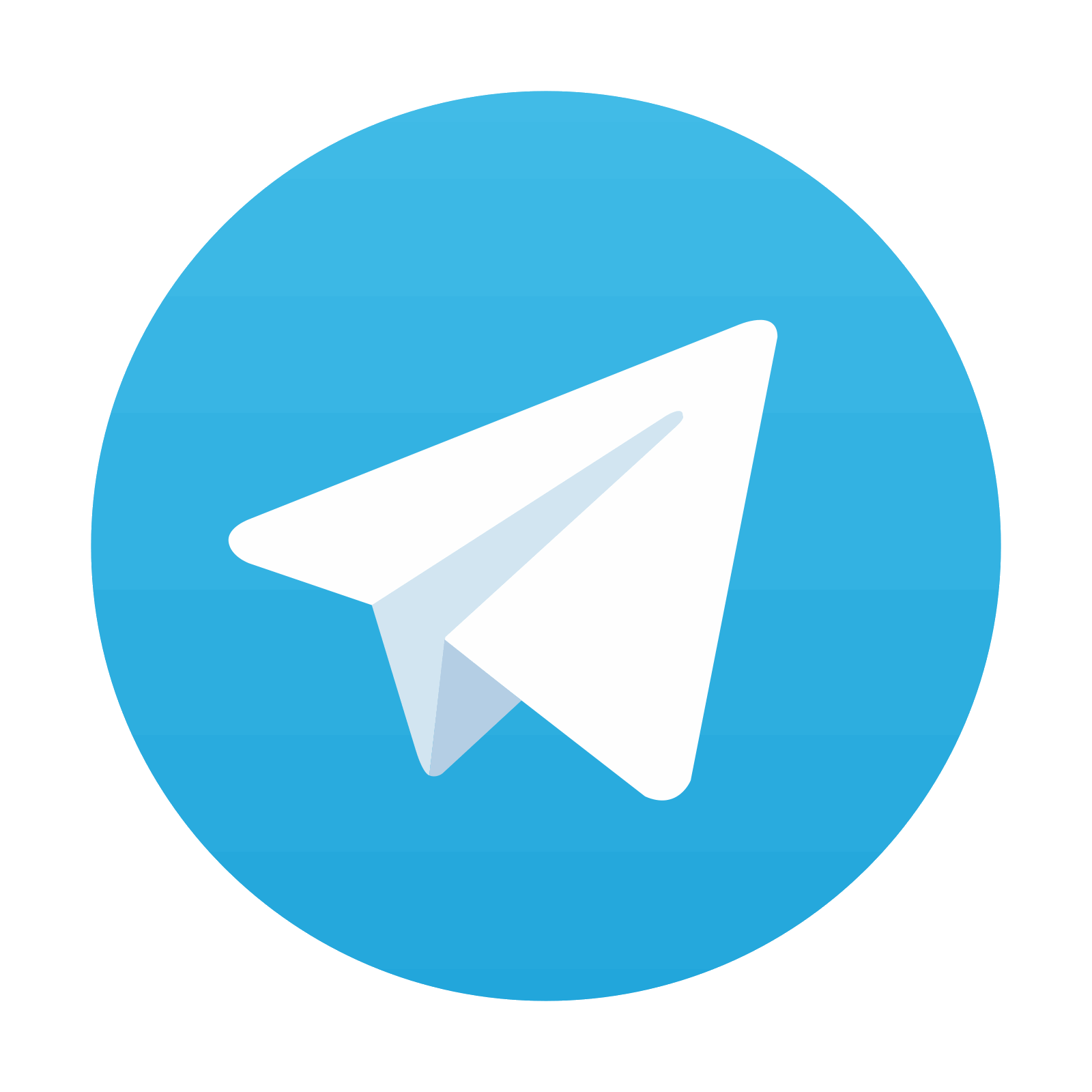
Stay updated, free articles. Join our Telegram channel
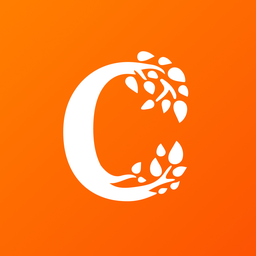
Full access? Get Clinical Tree
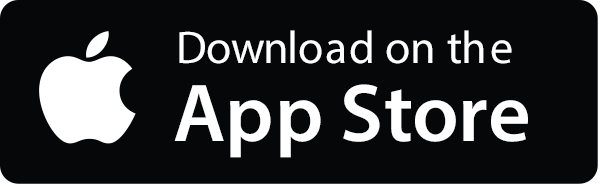
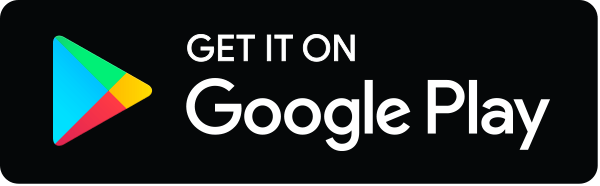