Cardiovascular Anatomy and Physiology
Glenn Woodworth Asish Das
Valerie Sera
▪ INTRODUCTION
Anesthesia and surgery is a delicate dance with the cardiovascular system. The majority of anesthetics interact with the cardiovascular system by depressing sympathetic outflow, dilating blood vessels, or directly interacting with the heart. Surgical stimuli, blood loss, and anesthetic procedures have the further ability to interact with the patient’s cardiovascular system. This chapter introduces the anesthesia technician to the fundamental anatomy and physiology of this important body system.
▪ SURFACE ANATOMY OF THE HEART
The heart is a set of hollow muscular pumps combined into a single organ. It is located in the middle of the chest (the mediastinum) between the lungs and their pleural coverings. It is shaped like an upside down pyramid that is tilted to the patient’s left. The base of the heart lies superiorly, and the apex lies inferiorly and leftward (Fig. 7.1). The heart itself is covered in a thick sac called the pericardium.
The heart is divided into two sides, a right and a left, and consists of a total of four chambers. The upper two chambers comprise the right and left atria, while the lower chambers are termed the right and left ventricles. The inferior and superior vena cava are connected to the right atrium (RA), and the pulmonary artery is connected to the right ventricle (RV). On the left side of the heart, the pulmonary veins are connected to the left atrium, and the aorta is connected to the left ventricle (LV) (Fig. 7.2). The heart is rotated slightly to the left, and the apex is tilted slightly anteriorly. Thus, the RA is anterior to the left atrium (LA), and the RV forms most of the anterior aspect of the ventricular mass. The LV is positioned on the left side of the heart, but because of the rotation and the tilting, it is also positioned slightly posterior and inferior to the RV. An understanding of the position of the heart is important to properly read the electrical signals that are generated by the heart and recorded on an electrocardiogram (ECG). The ECG is discussed in more detail later in this chapter.
▪ FETAL DEVELOPMENT
Major organ development occurs between the 4th and 8th week of fetal life. The heart is the first organ to complete its development during fetal growth. The heart begins as a primitive vascular tube that curves back on itself and bends anteriorly and rightward to form three distinct portions called the single-chambered primitive atrium, a ventricle, and the truncus. These develop further into a right heart and a left heart, atria and ventricles with intervening atrioventricular (AV) valves, and the aortic and pulmonary trunks. Errors in fetal development of the heart can result in significant malformations. For example, the heart may fail to form two ventricles, or the aorta and pulmonary trunks may be fused into a single trunk arising from both ventricles. An average adult heart is 12 cm from base to apex, 8-9 cm at its broadest transverse diameter, and 6 cm anteroposteriorly. Its weight varies with average 300 g in male and 250 g in females, with adult weight achieved between the ages of 17 and 20 years.
▪ PERICARDIUM
The heart is enclosed in a three-layered sac called the pericardium. The outer fibrous layer of the pericardium is attached to the great vessels, the sternum, and the diaphragm. It secures the heart to the sternum anteriorly and to the diaphragm inferiorly. The inner serous layer
consists of a visceral and parietal portion. The visceral pericardium, also known as the epicardium, covers the entire heart and great vessels. The parietal pericardium forms an inner lining to the fibrous pericardium. The space between the two layers of the serous pericardium is called the pericardial space. Normally, the pericardial space contains 30-50 mL of serous fluid that acts as a lubricant to decrease friction while the heart beats. The fibrous layer of the pericardium is noncompliant (not easily expandable) and helps to prevent dilation of the heart and restrict cardiac filling beyond the normal range. When there is an acute collection of fluid or blood in the pericardial space, a clinical condition called cardiac tamponade develops. Rapidly accumulating fluid in the pericardial space can put pressure on the heart chambers, severely restricting blood from entering the heart, and can result in circulatory collapse. Surgical intervention is often necessary to relieve cardiac tamponade.
consists of a visceral and parietal portion. The visceral pericardium, also known as the epicardium, covers the entire heart and great vessels. The parietal pericardium forms an inner lining to the fibrous pericardium. The space between the two layers of the serous pericardium is called the pericardial space. Normally, the pericardial space contains 30-50 mL of serous fluid that acts as a lubricant to decrease friction while the heart beats. The fibrous layer of the pericardium is noncompliant (not easily expandable) and helps to prevent dilation of the heart and restrict cardiac filling beyond the normal range. When there is an acute collection of fluid or blood in the pericardial space, a clinical condition called cardiac tamponade develops. Rapidly accumulating fluid in the pericardial space can put pressure on the heart chambers, severely restricting blood from entering the heart, and can result in circulatory collapse. Surgical intervention is often necessary to relieve cardiac tamponade.
▪ MYOCARDIUM
The heart wall consists of three layers. The outermost layer of the heart is the epicardium, consisting of epithelial cells that form a serous membrane that covers the entire heart. The innermost layer of the heart is the endocardium. It lines the inner surface of the heart, its valves, the papillary muscles, and the fibrous cords that connect the valves and continues into the major cardiac veins and arteries. The middle layer of the heart is the muscular layer known as the myocardium. It is responsible for the contractile action of the atria and ventricles. The human body contains three types of muscle: cardiac muscle, smooth muscle (found in other hollow organs like the bowel or bronchi), and skeletal muscle. Cardiac muscle cells are specialized muscle cells with amazing resiliency and capabilities. At an average heart rate (HR) of 60 beats per minute, the heart beats 3 billion or more times without resting in one’s lifetime. To accomplish this incredible feat, myocardial cells function in a slightly different manner than do skeletal muscle cells. Myocardial cells are rich in mitochondria, the energy engine of the cell. The large number of mitochondria makes the cells resistant to fatigue. This allows myocardial cells to perform almost continuous work as long as they have a rich supply of oxygen and nutrients. Unlike skeletal muscle, myocardial cells rapidly become unable to function if their blood supply is interrupted. In addition to extra mitochondria, myocardial cells have other unique properties. For example, they have the intrinsic ability to contract in the absence of stimuli in a rhythmic manner and have the ability to conduct electrical impulses from one myocardial muscle cell to the next. Both of these functions play an important role in the cardiac conduction system described below.
▪ CARDIAC CHAMBERS AND THE CIRCULATION OF BLOOD
As described above, the heart has four chambers: the RA, the RV, the LA, and the LV. The purpose of the heart is to circulate blood throughout the body where it can deliver oxygen and other nutrients, as well as pick up waste products like carbon dioxide. The blood circulates through the body in two loops or systems: the pulmonary circulation and the systemic circulation (Fig. 7.3).
Beginning with the terminal portion of the systemic circulation, blood is drained into the venous
system from the various organs and extremities. Blood from the lower portion of the body eventually drains into a large vein, the inferior vena cava (IVC). Blood from the upper portion of the body, including the upper extremities and the head and neck, drains into the superior vena cava (SVC). This venous blood has had much of its oxygen removed (deoxygenated) by the tissues
and contains extra waste products like additional carbon dioxide (CO2). The systemic venous blood from both large veins drains into the RA. Contraction of the RA pushes the deoxygenated blood through the tricuspid valve into the RV. Contraction of the RV forces the blood forward past the pulmonic valve into the pulmonary artery, the beginning of the pulmonary circulation. The pulmonary arteries branch into ever smaller arteries and arterioles before turning into capillaries. Capillary blood flows close to the pulmonary alveoli. The blood can now add oxygen from the alveoli and discharge CO2 into the alveoli (see Chapter 11). Once the blood has been oxygenated and discharged some of its CO2, it begins to collect from the capillaries into venules and eventually into the large pulmonary veins. The four large pulmonary veins drain into the left side of the heart and the LA. Contraction of the LA forces the oxygenated blood forward through the mitral valve and into the LV. The forceful contraction of the LV can generate high pressures, and the blood is ejected out of the LV through the aortic valve and into the aorta. From the aorta, the blood flows out to the systemic circulation, where it can perfuse the body. The blood can then return to the heart to complete the two loops. It is the continuous forward pumping action of the heart that keeps blood flowing through the body where it can deliver nutrients and pick up waste products.
system from the various organs and extremities. Blood from the lower portion of the body eventually drains into a large vein, the inferior vena cava (IVC). Blood from the upper portion of the body, including the upper extremities and the head and neck, drains into the superior vena cava (SVC). This venous blood has had much of its oxygen removed (deoxygenated) by the tissues
and contains extra waste products like additional carbon dioxide (CO2). The systemic venous blood from both large veins drains into the RA. Contraction of the RA pushes the deoxygenated blood through the tricuspid valve into the RV. Contraction of the RV forces the blood forward past the pulmonic valve into the pulmonary artery, the beginning of the pulmonary circulation. The pulmonary arteries branch into ever smaller arteries and arterioles before turning into capillaries. Capillary blood flows close to the pulmonary alveoli. The blood can now add oxygen from the alveoli and discharge CO2 into the alveoli (see Chapter 11). Once the blood has been oxygenated and discharged some of its CO2, it begins to collect from the capillaries into venules and eventually into the large pulmonary veins. The four large pulmonary veins drain into the left side of the heart and the LA. Contraction of the LA forces the oxygenated blood forward through the mitral valve and into the LV. The forceful contraction of the LV can generate high pressures, and the blood is ejected out of the LV through the aortic valve and into the aorta. From the aorta, the blood flows out to the systemic circulation, where it can perfuse the body. The blood can then return to the heart to complete the two loops. It is the continuous forward pumping action of the heart that keeps blood flowing through the body where it can deliver nutrients and pick up waste products.
![]() ▪ FIGURE 7.2 A coronal cut through the heart demonstrating the four chambers of the heart and their vascular connections. |
![]() ▪ FIGURE 7.3 Blood circulates through the heart in two loops forming the pulmonary and systemic circulations. |
Right Atrium
The RA is a thin-walled muscular chamber. It receives deoxygenated venous blood from the SVC, the IVC, and the coronary sinus. The coronary sinus empties into the RA just above the
tricuspid valve. In addition to the main chamber of the atrium, the RA has a small muscular pouch, the right atrial appendage. The RA is separated from the LA by the septum. The septal wall has an oval depression, the fossa ovalis. There are no true one-way valves in the venae cavae; thus, when the right atrial pressure rises, elevated pressure is reflected into the IVC and the SVC. Venous distention and systemic venous congestion are commonly seen when pressures are elevated in the heart as in congestive heart failure. Normal right atrial pressure ranges from 2 to 10 mm Hg.
tricuspid valve. In addition to the main chamber of the atrium, the RA has a small muscular pouch, the right atrial appendage. The RA is separated from the LA by the septum. The septal wall has an oval depression, the fossa ovalis. There are no true one-way valves in the venae cavae; thus, when the right atrial pressure rises, elevated pressure is reflected into the IVC and the SVC. Venous distention and systemic venous congestion are commonly seen when pressures are elevated in the heart as in congestive heart failure. Normal right atrial pressure ranges from 2 to 10 mm Hg.
Right Ventricle
The RV extends from the tricuspid valve nearly to the apex of the heart. The tricuspid valve prevents flow of blood backward from the RV into the RA. At the base of the heart, the RV extends to the left to form the infundibulum. The pulmonary valve prevents the flow of blood from the pulmonary artery backward into the RV. In addition to the main muscular walls of the RV, the RV contains two major papillary muscles and a third smaller papillary muscle. The papillary muscles connect the chordae, fibrous collagenous cords, to the leaves (cusps) of the tricuspid valve. The RV receives blood from the RA through the tricuspid valve and ejects it through the pulmonic valve into the pulmonary artery where it can flow to the lungs. The resistance to flow in the pulmonary circulation is approximately 1/10th that of the systemic circulation. Therefore, the RV does not need to generate as much pressure to pump blood to the lungs as the LV needs to pump blood to the body. This also explains why the RV muscle is much thinner than the thick muscular wall of the LV. Systolic pressure in the RV ranges from 15 to 25 mm Hg with end-diastolic pressures from 0 to 8 mm Hg (the concepts of systolic and diastolic pressures are discussed in detail below).
Left Atrium
The LA is the smaller of the two atria but has thicker walls. Its cavity and walls are mostly formed by the proximal part of the pulmonary veins, which are incorporated into the atria during fetal development. The left atrial aspect of the septum between the atria has a rough appearance and marks the site of the foramen ovale. In fetal life, the foramen remains open and is essential for fetal circulation. At birth, this foramen closes spontaneously, but in about 20%-30% of the normal population, the defect may persist without any symptoms. Although asymptomatic, a patent foramen ovale presents a potential passageway for gas bubbles or debris to pass from the right side of the heart to the left side without passing through the lungs. Under normal circumstances, most debris, like small blood clots or gas bubbles, will be stopped by the pulmonary microcirculation before they can reach the left side of the heart. Under abnormal circumstances (i.e., conditions in which the right atrial pressure is elevated), passage of gas or debris from the RA across the foramen ovale to the LA becomes a real possibility. This is important as any debris or bubbles in the left side of the heart could flow out of the heart and directly into the brain, causing a stroke. The LA receives oxygenated blood from the lungs through pulmonary veins. Normal filling pressure ranges from 4 to 12 mm Hg. Like the RA, the LA also has an appendage. The LA appendage forms a portion of the left heart border as seen on a chest x-ray. In atrial fibrillation (disorganized electrical activity of the heart), it can be a source of intracardiac blood clots. These clots can embolize systemically, causing a stroke or limb ischemia. When the atria fibrillates, it loses its contractile function and the left atrial appendage is less able to empty blood into the atrial main cavity. Blood pooling in the atrial appendage is prone to clotting.
Left Ventricle
The LV wall is almost three times thicker than the RV wall. It is designed to be a powerful contractile chamber that can maintain flow in the high-pressured systemic arteries. The LV cavity extends from the AV groove to the cardiac apex. The mitral valve forms the inlet to the LV. The outlet region is formed by the aortic valve. The septum dividing the right and left ventricles is thick and is functionally more a part of the LV than the RV (Fig. 7.4). A ventricular septal defect is the most common congenital heart defect in children younger than 2 years of age. Most small defects close spontaneously. Only the larger defects need surgical correction. The normal thickness of the LV wall is between 0.8 mm and 1.1 cm. In hypertrophic cardiomyopathy, the septum may get disproportionately thickened. When the ventricle contracts, the hypertrophic septum can obstruct the outflow of blood from the ventricular cavity into the aorta (dynamic
subaortic obstruction). This clinical condition may cause sudden death and requires medical treatment. The LV receives blood from the LA through the mitral valve and ejects blood through the aortic valve to the systemic circulation via the aorta. The mitral valve prevents the flow of blood backward into the LA during contraction of the LV. There are several fibrous chords attached to papillary muscles in the ventricle that support the mitral valve cusps. These chordae are essential for the proper functioning of the mitral valve. Pressure in the LV is high during muscular contraction (“systole”) and equals systemic blood pressures. When the ventricle relaxes (“diastole”), the pressure falls. Normal end-diastolic pressures in the LV range from 4 to 12 mm Hg. The smooth left ventricular outflow tract ends at the cusps of the aortic valve. The cusps of the aortic valve are attached in part to the aortic wall and in part to the supporting ventricular structures. The aortic valve in its closed position has three coronary sinuses (out pouching between the aortic wall and the cusp).
subaortic obstruction). This clinical condition may cause sudden death and requires medical treatment. The LV receives blood from the LA through the mitral valve and ejects blood through the aortic valve to the systemic circulation via the aorta. The mitral valve prevents the flow of blood backward into the LA during contraction of the LV. There are several fibrous chords attached to papillary muscles in the ventricle that support the mitral valve cusps. These chordae are essential for the proper functioning of the mitral valve. Pressure in the LV is high during muscular contraction (“systole”) and equals systemic blood pressures. When the ventricle relaxes (“diastole”), the pressure falls. Normal end-diastolic pressures in the LV range from 4 to 12 mm Hg. The smooth left ventricular outflow tract ends at the cusps of the aortic valve. The cusps of the aortic valve are attached in part to the aortic wall and in part to the supporting ventricular structures. The aortic valve in its closed position has three coronary sinuses (out pouching between the aortic wall and the cusp).
Cardiac Skeleton
The heart’s fibrous skeleton, the “annulus fibrosus,” is a firm anchor to which most of the heart’s muscles and valves are attached. The annulus fibrosus gives structure to the heart and acts as an insulator. Because the annulus fibrosus is not made up of myocardial muscle cells it does not conduct electrical impulses from one region of the heart to another. This ensures that electrical impulses traveling from the atria myocardial cells to the ventricular myocardial cells must move through a specialized pathway, the AV node. This system helps the heart regulate electrical signaling to coordinate contraction of the atria and ventricles as well as to help prevent abnormal electrical heart rhythms (arrhythmias).
Cardiac Conduction System
Myocardial muscle cells differ from skeletal muscle cells because of their inherent ability to spontaneously depolarize and repolarize in a rhythmic fashion (automaticity). Ventricular muscle cells spontaneously depolarize at a lower frequency (30-40 beats per minute) than atrial muscle cells, but in the intact heart, both are synchronized to a more rapid rhythm, generated by pacemaker cells in the RA. The pacemaker cells, called the sinoatrial (SA) node, and the specialized myocardial cells, which conduct the electrical signals to synchronize the contraction of all myocardial cells, make up what is known as the “cardiac conduction system” (Fig. 7.5).
The SA node is located in the high RA near the junction of the SVC and the RA. These “pacemaker cells” spontaneously depolarize and initiate contraction of the myocardial cells in the atria. Because all myocardial cells can conduct the electrical impulse and cause adjacent myocardial cells to depolarize, the electrical depolarization and subsequent contraction of atrial cells spreads like a wave beginning at the SA node. Several other specialized myocardial conduction cells conduct the electrical impulse from the RA to the LA (Bachmann’s bundle). The depolarization must also be conducted from the atria to the ventricles and more importantly the timing of atrial and ventricular contractions synchronized (see “Cardiac Cycle” below). If the atria and ventricles contract simultaneously, blood would not flow from the atria into the ventricles. The speed of conduction of electrical impulses through normal atrial and ventricular cells is about 0.5 m/s. The speed of
conduction of the electrical signal is much faster than the time it takes for muscular contraction; thus, the electrical signal from the SA node would reach the ventricle rapidly, long before the atrium has contracted. This would cause near simultaneous contraction of the atria and ventricles.
conduction of the electrical signal is much faster than the time it takes for muscular contraction; thus, the electrical signal from the SA node would reach the ventricle rapidly, long before the atrium has contracted. This would cause near simultaneous contraction of the atria and ventricles.
In the normal functioning heart, this does not happen because the fibrous annular tissue that separates the atria from the ventricles does not conduct the electrical impulse. Instead the impulse must pass through a region of cells in the inferior posterior portion of the atrial septum called the AV node. This group of specialized myocardial cells conduct the impulse at 1/10th the speed of normal myocardial cells. This has the effect of slowing the electrical signal before reaching the ventricles. This gives the atria a chance to contract and eject blood into the relaxed ventricle before the ventricle begins its contraction. In order to efficiently eject blood and generate pressure within the ventricle, the septum and apex of the ventricles must contract first, followed by the base of the heart. This coordination of contraction would not occur if the depolarization wave spread from the AV node through the ventricles from one cell to another. The heart utilizes additional specialized myocardial cells to rapidly conduct the electrical signals to the different portions of the ventricle to achieve effective contraction and blood ejection. These cells conduct the electrical signal 10 times faster than normal myocardial cells. After leaving the AV node, the electrical signal passes through the bundle of His to reach the base of the heart. It then passes on the endocardial side of the interventricular septum using specialized myocardical conduction cells (Purkinje fibers). It then moves along the endocardial side of each of the ventricles from apex to base using right and left branches of Purkinje fibers. The SA node, Bachman bundle, the AV node, the bundle of His, and the Purkinje fibers make up the myocardial conduction system.
Wolff-Parkinson-White and other syndromes have abnormal electrical pathways in the heart that lead from the atria directly to the ventricles bypassing the AV node. These conditions can lead to very fast heartbeats (tachycardia) and even to life-threatening abnormal heart rhythms.
Wolff-Parkinson-White and other syndromes have abnormal electrical pathways in the heart that lead from the atria directly to the ventricles bypassing the AV node. These conditions can lead to very fast heartbeats (tachycardia) and even to life-threatening abnormal heart rhythms.
Coronary Arterial Supply
The right and left coronary arteries arise from the ascending aorta just above the aortic valve. The coronary arteries supply the capillaries of the myocardium with oxygenated blood. One might think that the LV would not need any arteries because it could obtain oxygen directly from the oxygenated blood that flows through the ventricular cavities. However, the walls of the ventricles are too thick to obtain sufficient oxygen or eliminate waste products, like carbon dioxide, by diffusion. Arteries branching into arterioles and then capillaries must do the job. The blood is then collected into veins, which drain into the coronary sinus. The left coronary artery (LCA) has two main branches: the left anterior descending (LAD) and the left circumflex (LCX) arteries (Fig. 7.6).
The right coronary artery (RCA) and the LCX course around the heart in opposite directions in the AV groove. These two arteries throw off branches, with the terminal portions of the arteries meeting on the posterior aspect of the heart at an important landmark known as the crux of the heart. At this point, either the RCA or the LCX supplies the posterior descending artery (PDA), which descends in the interventricular groove toward the apex of the heart. This terminal branch supplies the posterior and inferior parts of the heart. Right or left coronary dominance is determined by which artery supplies the PDA. Seventy percent of people are RCA dominant, 10%-15% are LCA dominant, and 10%-15% have mixed right and left dominance. This is an important anatomical fact as the PDA supplies the crux and the posterior third of the ventricular septum. The AV node is located at the crux and is nourished by the PDA. Obstruction of the blood supply to the AV node can cause malfunction of the
node and prevent electrical impulses from traveling from the atrium to the ventricle properly (AV block). The RCA supplies the RA, RV, and inferior wall of the LV (if right coronary dominant). The LAD runs in the anterior interventricular groove and supplies the anterior wall of the LV. Along its course, it gives off diagonal arteries, which supply the lateral LV wall, and septal arteries, which supply the anterior two-thirds of the ventricular septum. The LCX artery supplies the LA and the lateral and posterior walls of the LV. The LAD, LCX, and RCA are considered major arteries because of their large area of distribution. Blockage in the proximal portion in any of these arteries can cause a large amount of myocardial cell death (myocardial infarction) and can significantly affect the heart’s ability to contract.
node and prevent electrical impulses from traveling from the atrium to the ventricle properly (AV block). The RCA supplies the RA, RV, and inferior wall of the LV (if right coronary dominant). The LAD runs in the anterior interventricular groove and supplies the anterior wall of the LV. Along its course, it gives off diagonal arteries, which supply the lateral LV wall, and septal arteries, which supply the anterior two-thirds of the ventricular septum. The LCX artery supplies the LA and the lateral and posterior walls of the LV. The LAD, LCX, and RCA are considered major arteries because of their large area of distribution. Blockage in the proximal portion in any of these arteries can cause a large amount of myocardial cell death (myocardial infarction) and can significantly affect the heart’s ability to contract.
Coronary Venous Circulation
The venous system of the heart consists of the thebesian veins, the anterior cardiac veins, and the coronary sinus (Fig. 7.7). The coronary sinus is located in the posterior AV groove near the crux and collects about 85% of the blood from the LV. It opens into the RA at the coronary sinus ostium near the orifice of the IVC. During cardiac surgery, the coronary sinus must often be cannulated. Anomalies in coronary sinus anatomy can present significant challenges for both the anesthesiologist and the cardiac surgeon.
Innervation
The heart is richly innervated by the autonomic nervous system to provide control of HR and the force of cardiac contractions (see Chapter 14).
Autonomic influence on the heart can modify cardiac function appropriately to meet the changing supply and demand of the body for blood flow. All portions of the heart are richly innervated by sympathetic fibers. When active, these sympathetic nerves release norepinephrine to act on the myocardial cells. Norepinephrine binds to β1-adrenergic receptors on cardiac muscle cells to increase the HR (chronotropy), increase the conduction velocity of electrical signals (dromotropy), increase the force of contraction (inotropy), and increase the speed of contraction and relaxation (lusitropy). Cholinergic parasympathetic innervation to the heart arises from the right and left vagal nerves and innervates the SA node, the atria, and the AV node. When active, these parasympathetic nerves release acetylcholine to act on myocardial cells. Acetylcholine interacts with muscarinic receptors on these cells to decrease the HR (SA node) and decrease the velocity of electrical signals moving through the AV node. Parasympathetic nerves may also act to decrease the force of contraction of atrial (not ventricular) muscle cells. Overall, parasympathetic activation acts to decrease cardiac pumping.
Autonomic influence on the heart can modify cardiac function appropriately to meet the changing supply and demand of the body for blood flow. All portions of the heart are richly innervated by sympathetic fibers. When active, these sympathetic nerves release norepinephrine to act on the myocardial cells. Norepinephrine binds to β1-adrenergic receptors on cardiac muscle cells to increase the HR (chronotropy), increase the conduction velocity of electrical signals (dromotropy), increase the force of contraction (inotropy), and increase the speed of contraction and relaxation (lusitropy). Cholinergic parasympathetic innervation to the heart arises from the right and left vagal nerves and innervates the SA node, the atria, and the AV node. When active, these parasympathetic nerves release acetylcholine to act on myocardial cells. Acetylcholine interacts with muscarinic receptors on these cells to decrease the HR (SA node) and decrease the velocity of electrical signals moving through the AV node. Parasympathetic nerves may also act to decrease the force of contraction of atrial (not ventricular) muscle cells. Overall, parasympathetic activation acts to decrease cardiac pumping.
▪ CARDIAC PHYSIOLOGY
Cardiac Action Potential
Like skeletal muscle, myocardial cells are able to contract due to the interaction of cellular proteins, actin and myosin. This interaction and subsequent contraction is dependent upon calcium for it to occur. As discussed above, the initiation of normal myocardial contraction begins with an electrical signal from the SA node. The electrical signal travels through the myocardial conduction system before reaching the rest of the myocardium. Upon reaching the myocardial cells, the electrical signal depolarizes the cell membrane, which opens channels to allow extracellular sodium and calcium to flow into the cell (Fig. 7.8). The inward flow of calcium causes additional calcium stores within the cell to be released. The calcium causes the cell to contract by promoting a temporary binding between actin and myosin. The calcium must be actively sequestered back into storage units within the cell to allow the muscle cell to relax. In addition, the myocardial membrane must be repolarized to prepare for another electrical signal. This sequence of events is called the cardiac action potential.
![]() ▪ FIGURE 7.8 The cardiac action potential demonstrates cell membrane potential changes over time caused by changing flows of ions into and out of the cell. A) standard action potential. B) action potential from a cell with automaticity-automatic depolarization. Note how phase 4 shows the cell slowly depolarizing until it reaches a threshold to initiate phase 0. (From Topol EJ, Califf RM, et al. Textbook of Cardiovascular Medicine. 3rd ed. Philadelphia, PA: Lippincott Williams & Wilkins; 2006.) |
The relative ratios of ions (molecules with strong positive or negative charges) like sodium, potassium, and calcium, inside and outside of the myocardial cell membrane create small electrical potentials or voltages across the membrane. For example, if there are more net positive ions outside of the cell than inside the cell, the cell would have a negative potential (the cell is polarized). By custom, the “positive” or “negative” potential of a cell is determined by the charge of the interior of the cell compared to the outside. At rest, myocardial cells have a negative potential called the resting membrane potential. Because of the charge differential between the inside and outside of the cell, the resting myocardial cell is a minibattery. Ions are kept inside and outside of the cell because the cell membrane is not permeable to ions. If an appropriate cellular channel for that ion is open, ions will flow into or out of the cell, depending upon the charge and concentration gradients. Once a sufficient electrical signal reaches the myocardial cell, it triggers a sequence of actions that open and close ion channels. The flow of ions across the channels causes the cell membrane potential to change over time resulting in the cardiac action potential. The phases of the cardiac action potential are as follows (Fig. 7.8):
Phase 4: Resting membrane potential
Phase 0: An electrical signal has caused the cell to open sodium channels and sodium rushes into the cell, depolarizing the membrane.
Phase 1: Fast sodium channels close. Small movements of potassium and chloride cause a slight dip in the membrane potential.
Phase 2: The membrane is kept depolarized by the inward flow of calcium ions balanced by an outward flow of potassium.
Phase 3: Repolarization occurs as the calcium channels close, but potassium continues to flow out of the cell.
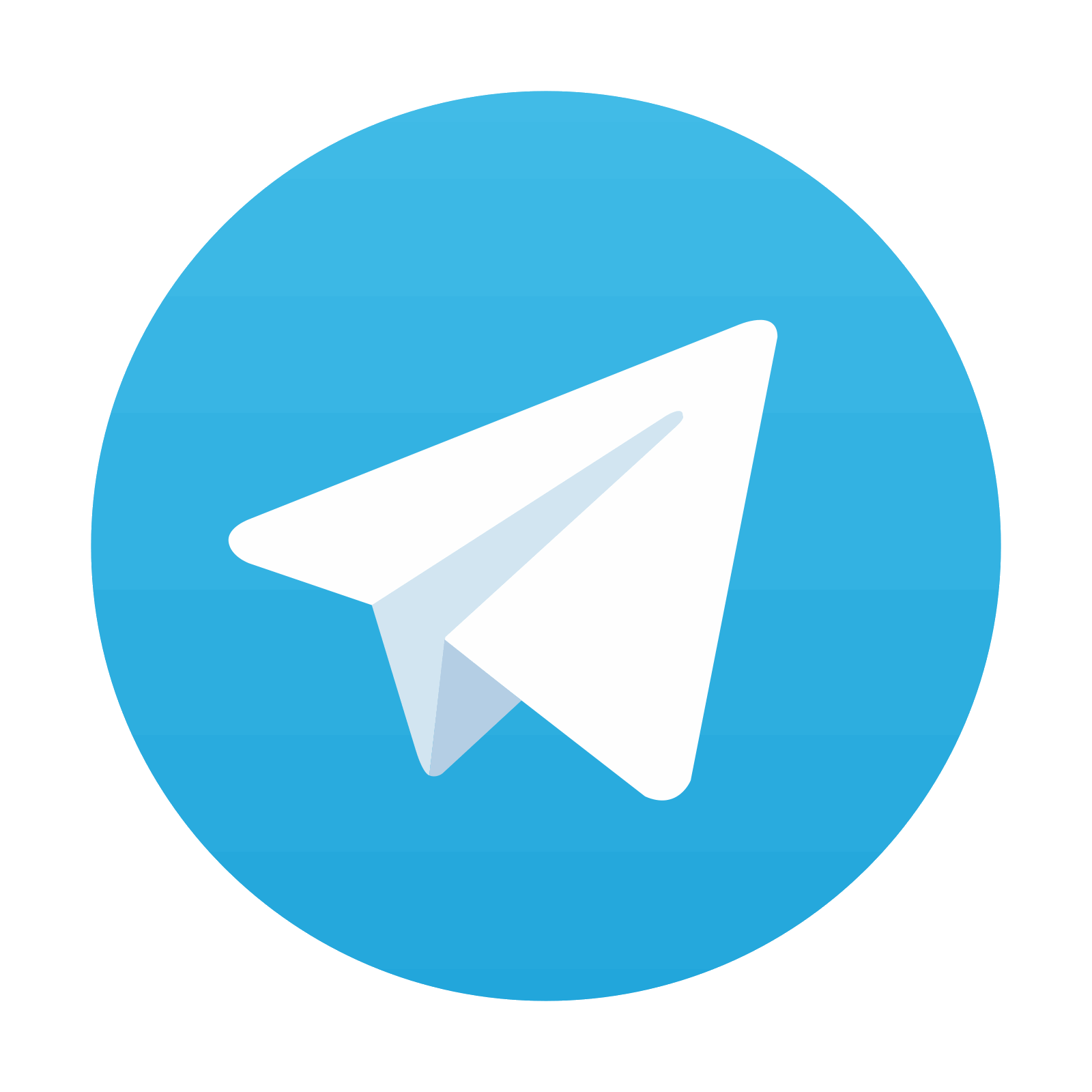
Stay updated, free articles. Join our Telegram channel
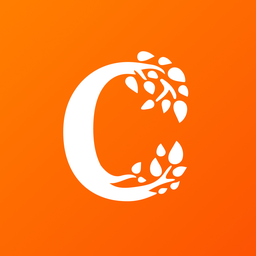
Full access? Get Clinical Tree
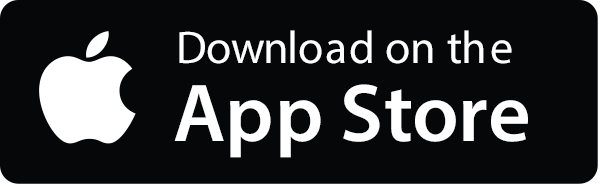
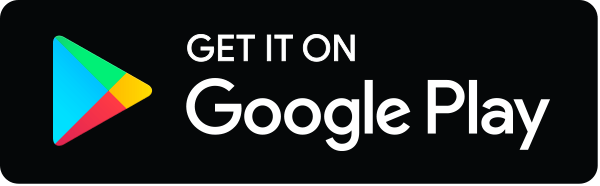