Introduction and history
In 1883, Sydney Ringer showed that calcium was essential for myocardial contraction ( ) . In 1934, Franklin McLean and Baird Hastings published a report in the Journal of Biological Chemistry showing that the ionized calcium concentration was proportional to the amplitude of frog heart contraction, whereas protein-bound and citrate-bound calcium had no effect ( ) . After many attempts to develop improved methodology, reliable ion-selective electrodes for measuring ionized calcium were ultimately developed and are now available.
The electrolytes calcium, magnesium, and phosphate have many roles in the structure and function of bone, the function of membranes, and the activation of numerous enzymes involved in genetic regulation, muscle contraction, and energy utilization. Parathyroid hormone, vitamin D, and calcitonin regulate the absorption, distribution, and excretion of these ions within the intestines, kidneys, bones, and soft tissues.
Other than diagnosing parathyroid dysfunction and hypercalcemia of malignancies, the usefulness of calcium measurements in diagnostic tests was of moderate interest through the 1970s. Over the past 40 years, a prominent role has evolved for monitoring calcium concentrations during certain surgeries and in critically ill patients.
Most calcium disorders are related to the following, for which calcium measurements are most useful:
- •
A deficiency of, or a defect in, parathyroid or vitamin D metabolism
- •
Malignancy, especially with hypercalcemia
- •
Kidney disease
- •
Magnesium deficiency
- •
Evaluating patients in critical illness, such as sepsis
- •
Evaluating neonates with unresolved hypocalcemia
- •
Excess administration of citrate, calcium, or saline
In addition, understanding the regulation of calcium and other electrolytes by parathyroid hormone (PTH), vitamin D, and calcitonin is helpful in the differential diagnosis of many calcium disorders.
A most important point to make is that the correction of hypocalcemia is not always necessary or warranted. Abnormal plasma calcium concentrations are often a marker for the disease severity and resolve spontaneously with a resolution of the primary disease process. Furthermore, low ionized calcium concentrations may be protective, such that correction may actually be harmful ( ) .
Calcium physiology
Approximately 99% of body calcium resides in the skeleton, of which a small percentage is freely exchangeable with the extracellular calcium. Although only 1% of the calcium in the body is present in the extracellular and intracellular spaces, the exchange and balance of calcium ions are critical in these spaces. The calcium concentrations in blood plasma are tightly regulated, with calcium ions playing a vital role in nerve impulse transmission, muscular contraction, blood coagulation, hormone secretion, and intercellular adhesion ( ) .
Overall calcium balance is regulated by the concerted actions of calcium absorption in the intestine, reabsorption in the kidney, and exchange from bone. All of these processes are controlled by hormones that are part of the complex mechanism regulating both the total body and extracellular calcium.
The normal plasma concentration of total calcium is approximately 2.20–2.55 mmol/L. While all calcium in blood plasma are calcium ions, much of these ions are bound to anions, with about 40%–45% bound to proteins (mostly albumin), and about 10%–15% complexed to smaller anions, mostly bicarbonate, citrate, lactate, and phosphate. The remaining 45%–50% are free calcium ions ( , ) .
The concentration of calcium in the cytoplasm of cells is about 15,000–20,000 times lower than the concentrations in extracellular plasma ( ) . This extracellular-to-intracellular gradient is maintained by the high-energy phosphates powering various ion pumps. The ionized calcium in the blood is the physiologically active portion of calcium and controls many neuromuscular and secretory processes ( ) .
As an example, ionized calcium plays a vital role in cardiovascular function. The inward flow of calcium ions into cardiac cells helps control cardiac rhythm by binding to contractile proteins in myocardial cells, which initiates the contractile process. The rate of flow of calcium ions into smooth muscle cells influences the tension of arterioles that regulate blood pressure. Calcium channels in the cell membrane regulate this flow by opening when the membrane is depolarized. Magnesium ions help stabilize the calcium channels. Cardiotropic drugs such as epinephrine and isoproterenol facilitate the transport of calcium ions through these channels promoting more rapid or stronger contractions increasing cardiac work. Calcium channel blockers, such as nifedipine, verapamil, and diltiazem lower blood pressure by relaxing blood vessels and slowing the heart rate which increases blood flow and oxygen supply to the heart and reduces cardiac work ( , ) .
Calcium ions enter cells through calcium channels, triggering the release of calcium ions from both the sarcoplasmic reticulum and the inner cell membrane. Regulation of calcium entry through these voltage-gated channels is essential for the control of physiological processes such as secretion, synaptic transmission, and excitation–contraction coupling ( ) . ATPases remove the excess calcium ions from inside the cell and transport calcium ions back into the sarcoplasmic reticulum for future release. In addition to these voltage-dependent calcium channels, other phosphorylation-dependent calcium channels are opened by a protein kinase activated by cyclic adenosine monophosphate (cAMP) ( ) . Several substances affect these channels: the cardiotropic drugs epinephrine and isoproterenol facilitate the transport of calcium ions; acetylcholine and calcium channel blockers hinder the transport of calcium ions ( ) .
Calcium ions are also important as second messengers. After stimulation at the cell surface by a specific molecule (first messenger), the inward flux of calcium ions (second messenger) initiates cellular actions, such as the production of hormones, such as insulin, aldosterone, vasopressin, and renin. This is done by keeping cytoplasmic Ca 2+ concentration low at rest and by mobilizing Ca 2+ in response to stimulus, which in turn activates the cellular reaction ( ) .
Regulation in the blood
Intestinal (GI) absorption of calcium . Most GI absorption of calcium is in the duodenum, jejunum, and ileum. Absorption occurs by either paracellular pathways through “tight junction” channels between cells, or transcellular pathways through cells. The paracellular route is partly regulated by 1,25-dihydroxy vitamin D (calcitriol) that increases the permeability of these tight junctions to Ca ions. Calcitriol also stimulates the intestinal cells to increase the synthesis of calbindin, an intracellular protein that accepts Ca ions from the intestinal microvilli, then ultimately releases the Ca ions into blood ( ) .
Renal regulation of calcium . As shown in Fig. 5.1 , 60%–70% of filtered Ca ions are reabsorbed in the proximal convoluted tubule (PCT), mostly by passive mechanisms, but small amounts are reabsorbed by active mechanisms primarily regulated by PTH and calcitonin ( ) . In the thick ascending limb of the loop (TAL), paracellular Ca ion absorption is driven by the Na–K–2Cl (NKCC2) cotransporter and the renal outer medullary K ion (ROMK) channel. Cotransporters are carrier proteins that simultaneously transport two different molecules or ions from one side of a membrane to the other at a rate of several thousand molecules per second. By using a favorable electrical or concentration gradient to transport one ion across a membrane, another ion is transported against its concentration gradient. The NKCC2 and ROMK processes transport Na, K, and Cl ions in a manner that yields a net positive charge in the lumen of the TAL, which drives the reabsorption of Ca ions by a paracellular route.

The cells in the TAL also express several “tight junction” proteins called claudins. Claudins are membrane proteins in these tight junctions between cells that regulate the flow of molecules and ions through these paracellular (between-cell) spaces. In the TAL, claudins reabsorb a small but important amount of Ca ions. Claudins are closely regulated by the calcium-sensing receptors (CaSR) that inhibit their ability to reabsorb Ca ions ( ) .
Hormonal regulation of calcium
Three hormones regulate serum calcium by altering their secretion rate in response to changes in ionized calcium: PTH, vitamin D, and calcitonin, although the role of calcitonin is less clear. Their actions are shown in Table 5.1 . A molecule with PTH-like actions, called PTH-related peptide (PTHrP), appears to have hypercalcemic actions similar to those of PTH, and may be especially important in hypercalcemia of malignancy ( , ) .
Hormone | Actions |
---|---|
PTH | Promotes renal tubular reabsorption of Ca ions by inhibiting the calcium sensing receptors |
Promotes urinary excretion of PO 4 | |
Activates 1-a-hydroxylase in the kidney, which synthesizes active vitamin D (calcitriol). Vitamin D activates GI absorption of calcium | |
Stimulates bone resorption that releases Ca ions into the ECF | |
Vitamin D (calcitriol) | Promotes intestinal absorption of Ca and PO 4 ions |
Promotes tubular reabsorption of Ca and PO 4 ions in the kidney | |
Regulates its own homeostasis by suppressing renal production of 1-α-hydroxylase and activating the production of 25-α-hydroxylase | |
Calcitonin | Is produced in the thyroid gland in response to hypercalcemia |
Blocks bone osteoclasts from releasing Ca ions into the ECF | |
Blocks Ca absorption in the kidney and GI tract |
Parathyroid hormone . When calcium levels in blood fall, cells in the parathyroid glands respond by synthesizing and secreting PTH, which acts synergistically with vitamin D to increase blood calcium levels. The parathyroid glands respond rapidly to a decrease in ionized calcium concentration in blood, with a fourfold increase in PTH secretion stimulated by a 5% decrease in ionized calcium ( , , ) and is stopped by an increase in ionized calcium ( , ). PTH is synthesized in the parathyroid gland as a precursor molecule of 115 amino acids. After cleavage in the gland, intact PTH is secreted into the blood, where it circulates as an intact hormone and also as amino-terminal, carboxy-terminal, and midmolecular fragments that have varying biological activity. Both intact PTH and amino-terminal PTH interact with PTH receptors on bone and renal cell membranes ( ). In bone, PTH activates osteoclasts to release cytokines that enhance the breakdown of bone to release calcium and phosphate. In kidneys, PTH conserves calcium by increasing tubular reabsorption of Ca ions and lowers phosphate by inhibiting tubular reabsorption of phosphate. PTH also activates a specific 1-α-hydroxylase enzyme in the kidney that increases the production of active vitamin D.
Vitamin D. Inactive forms of vitamin D 3 are obtained either from the diet or from exposure of skin to sunlight. These are converted in the liver to 25-hydroxycholecalciferol (25-OH-D 3 ), then activated by the enzyme 1-α-hydroxylase in the kidney to form 1,25-dihydroxycholecalciferol [1,25-(OH) 2 -D 3 ; calcitriol], the biologically active form. As noted earlier, PTH promotes this conversion to active vitamin D by activating the production of the 1-α-hydroxylase enzyme, and phosphate inhibits this production. The metabolism of vitamin D is shown in Fig. 5.2 .

Active vitamin D ( ) :
- •
Enhances the activity of calcium pumps and calcium channels in intestinal cells to increase calcium and phosphate absorption
- •
Has a short-term enhancement of PTH-activated bone resorption to release calcium into the blood
- •
Acts with PTH to minimize renal excretion of calcium
- •
Contributes to the negative feedback regulation of PTH through specific receptors for 1,25-(OH) 2 -D 3 on the parathyroid glands
Calcitonin . If calcium levels in the blood increase, several mechanisms act to reverse the increase: (1) C cells in the thyroid gland synthesize calcitonin, which exerts its calcium-lowering effect by inhibiting the osteoclasts in bone, which promotes deposition of calcium into bone and lowers calcium concentrations in blood. Calcitonin may also promote this effect by inhibiting the actions of both PTH and vitamin D on these cells. It is still not clear if calcitonin contributes to normal calcium homeostasis, because (a) persons without thyroid glands are still able to regulate their calcium levels in the blood, and (b) calcitonin does not change in response to small (1%–2%) increases in ionized calcium. A study on hemodialysis patients showed that ionized calcium had to increase by ∼10% to stimulate a calcitonin response ( , ) . Another study noted that a 0.4 mmol/L increase was required to stimulate a response ( ) .
Distribution in cells and blood
More than 99% of calcium in the body is in the bone as hydroxyapatite, a complex molecule of calcium and phosphate. The remaining 1% is mostly in the blood and extracellular fluid. The amount of intracellular calcium is much lower than in blood, and this is especially true of cardiac and smooth muscle cells, where the concentration of free ionized calcium in the cytosol is 10,000–20,000 times less than that of blood. Having this large gradient ensures the rapid inward flux of calcium ions necessary to trigger muscle contraction. Maintaining this gradient also requires efficient mechanisms for pumping out calcium ions. Loss of this very large gradient will lead to cell death ( ) .
Calcium in the blood is distributed among several forms: free calcium ions, bound to protein, and bound to smaller anions ( , ) . As shown in Fig. 5.3 , bound forms of calcium are in equilibrium with the free calcium ions. This distribution can change in many diseases, but major changes may occur during surgery or in critically ill patients because of changes in citrate, bicarbonate, lactate, and phosphate. These changes, in addition to the effect of pH on Ca 2+ binding to proteins, are the principal reasons why ionized calcium cannot be reliably calculated from total calcium measurements in acutely ill individuals. As a rough guide, pH changes influence the concentration of ionized calcium, with a decrease of 0.1 pH unit increasing ionized calcium by ∼0.05 mmol/L (0.2 mg/dL) ( ).

Hypocalcemia
Signs and symptoms of hypocalcemia
Hypocalcemia is considered to be a total calcium <8.5 mg/dL (<2.12 mmol/L) or an ionized calcium <1.00 mmol/L. As ionized calcium decreases, concentrations of 0.50–0.75 mmol/L may require administration of calcium and concentrations less than 0.50 mmol/L may produce tetany or life-threatening complications ( ) . Ionized calcium concentrations below 0.70 mmol/L have been associated with higher morbidity and mortality ( , ) .
Symptoms of hypocalcemia are often manifested as cardiovascular disorders such as cardiac insufficiency, hypotension, and arrhythmias. Hypocalcemia can also cause irregular muscle spasms, called tetany, and numbness around the mouth. The rate of fall in ionized calcium initiates symptoms as much as the absolute concentration of ionized calcium ( ) .
Causes of hypocalcemia
Prompt recognition of abnormal ionized calcium is especially important in patients with sepsis, seizures, arrhythmias, hypotension, heart failure, shock, or burns.
Hypocalcemia often occurs in patients with the following conditions ( ) :
- •
Chronic or acute kidney failure
- •
Magnesium deficiency
- •
Parathyroid gland insufficiency
- •
Acute pancreatitis
- •
Critical illnesses
- •
During surgery
- •
Blood transfusion
- •
Hepatic disease
- •
Hungry bone syndrome
- •
Neonates
These and other frequent causes of hypocalcemia are presented in Table 5.2 .
|
|
|
|
|
|
|
|
|
|
|
|
Kidney disease leading to vitamin D deficiency . Patients with kidney disease often have altered concentrations of calcium, phosphate, albumin, magnesium, and H + (pH). Both hyperphosphatemia and hypocalcemia are often features of renal disease, most likely due to complex alterations in the production and/or response to both PTH and vitamin D.
In chronic renal disease, secondary hyperparathyroidism often develops to compensate for hypocalcemia caused by diminished vitamin D production, due to hyperphosphatemia that inhibits synthesis of renal 1α-hydroxylase. Monitoring and controlling ionized calcium in renal disease and renal dialysis may avoid problems due to hypocalcemia, such as osteodystrophy, cardiovascular instability, or problems arising from hypercalcemia such as soft tissue calcifications ( , ) .
Hypomagnesemia . As hypomagnesemia in hospitalized patients has become more frequent, chronic hypomagnesemia has also become recognized as a frequent cause of hypocalcemia. Hypomagnesemia may cause hypocalcemia by:
- •
Inhibiting the glandular secretion of PTH across the parathyroid gland membrane ( , ).
- •
Impairing PTH action at its receptor site on bone ( ).
- •
Causing vitamin D resistance ( , ).
Hypoparathyroid conditions . The most common causes of hypoparathyroidism are due to either surgical removal of excess parathyroid tissue in parathyroid surgery or parathyroid hypofunction after thyroid surgery. This condition is usually transient (lasting <5 d) unless surgery has removed too much parathyroid tissue or has interfered with parathyroid blood supply. Serum calcium is also useful for monitoring after neck surgery until a rise in the ionized calcium indicates recovery of the parathyroid gland ( ) . Intraoperative PTH measurements are commonly done to ensure the appropriate amount of parathyroid tissue has been removed ( ). Primary hypoparathyroidism acquired as a genetic or autoimmune disorder is an uncommon condition.
Pseudohypoparathyroidism is characterized by end-organ resistance to PTH ( ) . In this condition, PTH binds to receptors on the kidney and other cells, but the cellular response to PTH is impaired, leading to elevated circulating concentrations of PTH. The defect may be in the guanine nucleotide regulatory protein Gs-alpha, which is required to activate cAMP in the normal cellular response to PTH. The combined effects of PTH resistance and hyperphosphatemia can lead to suppression of 1α-hydroxylase activity, producing a deficiency of active vitamin D and hypocalcemia ( , ) .
Acute pancreatitis . Many patients with acute pancreatitis develop hypocalcemia. While the standard mechanism often cited is the precipitation of calcium ions by free fatty acids generated during acute pancreatitis, the mechanism is certainly more complex. Parathyroid dysfunction, calcitonin release, and endotoxin release may play roles as well. A consistent cause appears to be diminished secretion of PTH, which may either be low or inappropriately normal for the degree of hypocalcemia ( , , ) .
Critical illness and sepsis . Critically ill patients with sepsis, thermal burns, renal failure, and/or cardiopulmonary insufficiency, frequently have symptoms attributable to hypocalcemia, such as seizures, arrhythmias, hypotension, heart failure, or shock ( ). These patients also tend to have abnormal acid–base regulation, diminished protein and albumin, and ionized hypocalcemia, which may directly affect mean arterial pressure ( , ) . Inflammatory mediators such as cytokines likely play a role in the development of these conditions.
Hypocalcemia is found in over 50% of patients in intensive care, although there are conflicting results about whether hypocalcemia should be corrected or not ( , ) . Some suggest that hypocalcemia is simply a marker of disease severity so that hypocalcemia will spontaneously resolve with resolution of the primary disease process ( , ) . Others report that if calcium is carefully administered to maintain normal ionized calcium concentrations in blood, adverse side effects are not observed ( ) .
During surgery . In patients undergoing surgery, adequate calcium concentrations enhance cardiac output and maintain blood pressure. Monitoring and adjusting calcium concentrations may be warranted in open-heart surgery when the heart is restarted, because normalizing ionized calcium by administering calcium is a prudent action to prevent the cardiac alterations associated with hypocalcemia ( ) .
Blood transfusion . Monitoring ionized calcium is important in patients receiving large volumes of citrated blood products, which may bind and reduce serum calcium concentrations. This can occur during surgery, trauma, or other causes of massive hemorrhage and is especially notable during liver transplantation when liver function (the major organ for metabolizing citrate) is compromised or absent.
Hepatic disease . More severe liver diseases that impair synthetic functions can cause vitamin D deficiency from impaired 25-hydroxylation of vitamin D, decreased production of bile salts that inhibit GI absorption of vitamin D, or decreased synthesis of vitamin D-binding protein ( ) .
Hungry bone syndrome . Hungry bone syndrome refers to hypocalcemia caused by the deposition of calcium into the bone at an accelerated rate during the healing of osteodystrophies. This syndrome is sometimes seen after surgical correction of long-standing secondary hyperparathyroidism by partial parathyroidectomy. It may also occur from osteoblastic metastases in various malignancies.
Neonatal hypocalcemia . Ionized calcium concentrations in the blood of apparently normal neonates are high at birth, rapidly decline by 10%–20% after 1–3 days, then stabilize at concentrations slightly higher than in adults after ∼1 week ( , ) . Hypocalcemia in neonates is defined as follows:
- •
Total calcium <2 mmol/L (8 mg/dL) in term infants or <1.75 mmol/L (7 mg/dL) in preterm infants.
- •
Ionized calcium from 0.75 to 1.10 mmol/L (3.0–4.4 mg/dL).
Signs are primarily neurologic and include irritability, muscle twitches or tremors, poor feeding, lethargy, and seizures in more severe cases ( ) .
Early-onset hypocalcemia is common and ordinarily resolves in a few days, and asymptomatic neonates with serum calcium levels >1.75 mmol/L (7 mg/dL) or ionized calcium >0.88 mmol/L (3.5 mg/dL) rarely require treatment. If total calcium concentrations in term infants are <1.75 mmol/L (7 mg/dL), or are <1.5 mmol/L (<6 mg/dL) in preterm infants, 10% calcium gluconate (200 mg/kg) may be given by slow IV infusion over 30 min. Too-rapid infusion can cause bradycardia, so heart rate should be monitored during the infusion ( ) .
Slightly lower concentrations of ionized calcium in healthy infants may be a normal physiological stimulus to activate parathyroid gland function and do not require treatment.
Factors that are related to the incidence and severity of hypocalcemia in neonates include ( , ) :
- •
Prematurity or infants who are small for gestational age
- •
Hypomagnesemia
- •
Maternal diabetes
- •
Complications during delivery
- •
Perinatal or birth asphyxia
A transient hypoparathyroidism may cause hypocalcemia in preterm neonates and some small-for-gestational-age neonates. In infants of mothers with diabetes or hyperparathyroidism, the mother’s higher plasma ionized calcium concentrations during pregnancy can cause a hypoparathyroid condition in the child. Perinatal asphyxia may also increase serum calcitonin, which inhibits calcium release from bone ( ) .
Laboratory evaluation of hypocalcemia
Total calcium, “corrected” total calcium, and ionized calcium
Although total calcium is often the initial laboratory test to detect hypocalcemia, ionized calcium is a more definitive parameter for confirming a calcium abnormality. Once hypocalcemia is confirmed with a low ionized calcium concentration, the sequence of laboratory tests to elucidate the cause is shown in Fig. 5.4 .
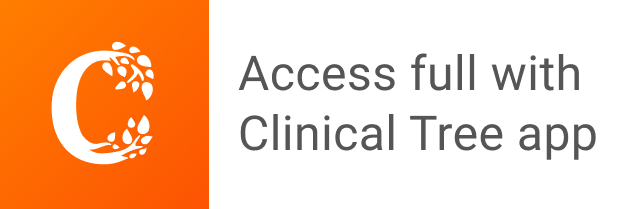