Blood Products and Transfusion Therapy
Karam O
Spinella Pc
Wong E
Luban N
KEY POINTS

Red Blood Cell Transfusion



Plasma Transfusion


Platelet Transfusion

BRIEF HISTORY OF TRANSFUSIONS
Perhaps the earliest recorded case of blood transfusion was that of Pope Innocent VIII (1432-1492). In April 1492, the Pope was in a coma after suffering a stroke and was given the blood of three boys through the mouth, as the concept of circulation was not yet discovered. Despite this treatment, the Pope (and all three boys) died. In 1628, William Harvey explained the circulatory system in humans. This knowledge permitted intravenous transfusion to be considered as a therapeutic option. In the early 1660s, Sir Christopher Wren used a quill-and-bladder syringe to inject fluid into the vein of a dog as a new method of administering medications. Richard Lower performed the first direct blood transfusion from one dog to another in 1665 by connecting an artery to a vein via a silver tube. In 1667, the French physician Jean Baptiste Denys used Lower’s technique to successfully transfuse the blood of sheep into both a 15-year-old boy and a woman with postpartum hemorrhage. However, in 1668, Denys’ fourth patient died after a transfusion and, although it was later established that the cause of death was arsenic poisoning by the patient’s wife, transfusions were banned first in France and England.
In 1818, James Blundell, an obstetrician at Guy’s Hospital in London, transfused a patient with blood taken from her husband’s arm with a syringe. He went on to perform 10 transfusions over the next 12 years, 5 of which were beneficial for the patients. In 1900, Karl Landsteiner described the concept of different blood groups. In 1907, George Crile published the “Technique of Direct Transfusion of Blood,” in which he described the interposition of a cannula between the donor’s artery and the receiver’s vein. In 1907, Ludvig Hektoen described hemolysis in incompatible transfusions, and in 1913, Ottenberg published the concept of blood group screening prior to transfusions. The major problem that remained was the preservation of blood, as it would coagulate if stored. In 1916, Rous and Turner published “The Preservation of Living Red Blood Cells in Vitro,” in which they described sodium citrate as an anticoagulant. One of their students, Oswald Robertson, was sent to the battlefields in Europe, and transfused wounded soldiers with whole blood stored for up to 26 days. He published his work in 1918.
In 1943, Loutit and Mollison discovered that adding an acid to stored blood stabilized the glucose at high temperatures and allowed the stored blood to be sterilized with heat to prevent the transmission of infectious diseases. Since then, the ability to separate whole blood into individual components, the transition from the use of bottles to that of plastic storage containers, and alterations of storage solutions increasing the storage duration (and therefore availability of blood products) led to modern era blood banking.
TRANSFUSION
Constitution of Red Blood Cell Transfusions
Red blood cell (RBC) units are processed from whole blood or from apheresis collections. There are many different processing methods, and there are some recent data suggesting that quality is affected by the method used. Most frequently, whole blood is collected at the donation site into a plastic bag containing an anticoagulant-preservative solution. As soon as possible, the whole blood is centrifuged, the supernatant is used to prepare other blood products (frozen plasma, platelets, gamma globulin, and albumin), and the RBCs are resuspended in another anticoagulant-preservative solution. However, RBC units still contain a significant proportion of plasma and in most solutions (ACD-A, citrate phosphate dextrose [CPD], CPDA-1), the final hematocrit of 60% ± 10%. In many countries, RBC units are filtered to reduce leukocytes prior to storage (see below). After standard leukoreduction, the white blood cell count is decreased from 109 to <1 × 106/unit of blood.
Scientific Foundation of Red Blood Cell Transfusion
Oxygen Transport
Oxygen transport requires extracting oxygen (O2) from the atmosphere and then delivering it to cells, where it can be used for essential metabolic processes. Although some cells can temporarily produce energy in the absence of oxygen (anaerobic metabolism), other organs (such as the brain) are made up of cells that rely on continuous oxygen supply for their metabolism (aerobic metabolism). Physiologically, oxygen delivery (DO2, in mL O2/min) can be described with the following formula:
DO2 = cardiac output × arterial O2 content
The formula for the arterial O2 content (CaO2) is
where CaO2 and PaO2 are expressed in millimeters of mercury (mm Hg), arterial saturation in oxygen (SaO2) is expressed as a fraction, and hemoglobin (Hb) concentration is expressed in grams per deciliter (g/dL).
Not all the oxygen is used for the metabolism, and some returns to the lungs. The amount of oxygen utilized by the cells can be calculated by the oxygen consumption ([V with dot above]O2) equation:
[V with dot above]O2 = cardiac output × 1.34 × Hb × (SaO2 – SVO2)
where arterial and venous saturations in oxygen (SaO2 and SVO2) are expressed as a fraction, and hemoglobin (Hb) concentration is expressed in grams per deciliter (g/dL).
Under normal circumstances, DO2 exceeds [V with dot above]O2, indicating a physiologic reserve of oxygen delivery in excess of tissue O2 requirements such that [V with dot above]O2 will remain constant even if there are mild to moderate reductions in DO2. If DO2 decreases progressively beyond a certain threshold, the physiologic DO2 reserve is exhausted, and thereafter [V with dot above]O2 decreases linearly with further decreases in DO2. This point in the DO2/[V with dot above]O2 relationship is called the “critical threshold” and indicates the point at which [V with dot above]O2 is no longer governed by the metabolic need of the tissues but rather becomes limited by O2 supply (DO2). As DO2 becomes inadequate, tissue hypoxemia occurs. Tissue hypoxia from low DO2 may be due to a low Hb concentration (anemic hypoxia), low cardiac output (stagnant hypoxia), or low Hb saturation (hypoxic hypoxia). This is illustrated in Figure 42.1. It is important to recognize that calculation of [V with dot above]O2 is an indirect measure, which is susceptible to mathematical coupling errors. Direct measure of [V with dot above]O2 such as with direct calorimetry is more accurate but also more difficult to perform.
Adaptation to Anemia. Anemia is defined as Hb concentrations below the “normal” range for age. Approximately 50% of critically ill children treated in typical North American PICUs are anemic (1). The major physiologic consequence of anemia is a reduction in the DO2 capacity of blood. The physiological adaptation to anemia is initially an increase in the other DO2 variables or a decrease in [V with dot above]O2. These processes include increased extraction of available O2, increased heart rate and stroke volume (i.e., cardiac output), redistribution of blood flow from nonvital organs toward the heart and brain (usually at the expense of the splanchnic vascular bed), and a shift to the left in the oxyhemoglobin dissociation curve (i.e., a decrease in O2 affinity).
Impairment in Adaptive Mechanisms. A number of diseases and host characteristics may impair the adaptive mechanisms to anemia in critically ill patients. The metabolic rate is increased, which decreases the DO2 reserve in systemic inflammatory response syndrome (SIRS), sepsis, and multiple-organ dysfunction syndrome (MODS). Patients with sepsis and MODS may also have impaired left ventricular function and poor regulation of vascular tone due to impaired nitric oxidemediated vasoregulation, restricting DO2 and redistribution of blood flow, respectively.
A number of host characteristics specific to children and infants may impair their adaptive mechanisms. The energy requirements of young infants are much higher than those in adults. This difference is mostly attributable to growth, and it means that they need more substrates, including O2 and nutrients. In addition to increased metabolic demands, major differences in O2 transport also exist between adults and children in the first year of life. Fetal Hb has a left-shifted O2 equilibrium curve, and the proportion of fetal Hb is significant during the first months of life. Physiologic anemia is expected during this period, partially explaining why the Hb concentration varies so much in the newborn and the infant. During the first weeks of life, myocardial compliance is decreased, significantly impairing diastolic filling, which limits increases in stroke volume. Moreover, in newborns and infants, the heart rate is already high, even at rest, which also limits the ability to increase cardiac output. Therefore, many characteristics specific to critically ill children alter their ability to physiologically adapt to low Hb concentrations.
Oxygen Kinetics and Transfusions. DO2 exceeds resting O2 requirements in healthy patients, but safety margins rapidly disappear during critical illness, where [V with dot above]O2 is often increased (e.g., burns, sepsis, ARDS) and DO2 is often decreased (e.g., shock, sepsis). Some investigators have shown that patients with sepsis do indeed have some DO2/[V with dot above]O2 dependence, which has led some to hypothesize that sepsis may increase the “critical threshold” DO2 at which tissue oxygen consumption becomes limited by O2 supply. The resultant tissue hypoxia contributes to the evolution of irreversible and often fatal MODS. However, RBC transfusion in critically ill patient groups has not been shown convincingly to increase DO2 to the point that [V with dot above]O2 is independent of DO2 and tissue hypoxia has been abolished. The potential reasons are multifactorial and may include (2) the following:
Impaired tissue O2 extraction in some critically ill patients;
Decreased microcirculatory flow from increased blood viscosity after transfusion.
Furthermore, the [V with dot above]O2 calculation uses data from the central circulation, not the microcirculation. But DO2 and [V with dot above]O2 occur in the microcirculation, and there is limited evidence that RBC transfusion improves either at the microcirculatory level. Therefore, it remains uncertain if RBC transfusion is the most useful method to achieve optimal DO2. The effect of RBC storage duration may influence RBC transfusion oxygen kinetics. The clinical relevance of these effects is controversial and currently under intense investigation.
Microcirculatory Effects of Native and Transfused Red Blood Cells
The effects of transfused RBCs on the distribution of systemic blood flow to specific organs have been described. Despite investigation, the effect of transfused RBCs on systemic DO2 is still controversial. For example, RBC transfusion may increase sublingual microcirculation without having an effect on global DO2 (3). It remains unclear how transfused RBCs influence DO2 in the microcirculation owing to the difficulty in obtaining in situ measurements of blood viscosity, microcirculatory flow, and [V with dot above]O2.
In theory, either too low or too high viscosity is associated with a risk of decreasing blood flow through small vessels. Capillaries collapse if the hematocrit is too low, and RBC transfusion may be viewed as a means of restoring blood viscosity (4). On the other hand, some have suggested that capillary resistance to blood flow increases rapidly if the hematocrit level increases (from 33% to 55%), which may result in microcirculatory stasis and impaired local DO2 to the tissues (5).
The microcirculatory effects of transfused RBCs may also be related to the generation of inflammatory mediators as some cytokines may mediate vasoconstriction or thrombosis of small vessels, causing local ischemia.
Transfused RBCs differ from their endogenous counterparts. During storage, numerous changes occur to RBCs and the unit supernatant; these changes progress over time. Detailed mechanisms of these changes are reviewed in detail elsewhere (6). To summarize, RBC energetics alter during storage (principally from impaired glycolysis), which results in an adverse impact upon antioxidant systems and an increase in iron-based free radical generation. Consequently, intraerythrocytic 2,3-DPG is diminished, NO bioavailability is reduced, the RBC membrane loses deformability, RBC adhesion and aggregation are increased, and RBC membrane microparticles are generated. Combined, these effects reduce both perfusion and oxygen delivery. Moreover, RBC membrane microparticles generated during storage have immune suppressive and procoagulant properties. While these effects of storage have been demonstrated, their clinical relevance is unclear. The two-hit hypothesis, as applied to RBC storage duration, suggests critical illness (a state of immune, endothelial, and hemostasis dysregulation) increases vulnerability to impact from RBCs altered by storage and that the likelihood of adverse clinical effects from transfusion progresses with storage duration. A large, multicenter randomized controlled trial (RCT) is planned to examine this question in critically ill children. In summary, while RBC transfusions increase circulating oxygen content, donor RBCs may paradoxically decrease O2 delivery to tissue, as well as disturb immune function and exacerbate thrombophilia in patients with critical illness, potentially increasing the risk of adverse clinical outcomes.
Hemolysis also occurs in packed RBC (PRBC) units over time. Free intravascular Hb as well as Hb within RBC membrane microvesicles may cause vasoconstriction, via the binding of NO. In summary, while RBC transfusions increase global DO2, microcirculatory flow and O2 availability may decrease in some tissues.
Immunologic Effects of Allogeneic Red Blood Cell Transfusions

The white blood cells (WBCs) and the cytokines in unfiltered packed RBCs may trigger or maintain an SIRS in the RBC recipient. Giving an RBC transfusion to a critically ill
patient with SIRS may stimulate their inflammatory syndrome, which may result in the development of MODS (second-hit theory of MODS). Some data suggest that the inflammatory risks of RBC transfusion decrease significantly if the packed RBC units are prestorage-leukocyte depleted. However, an in vitro study showed that even leukoreduced RBC units induced cytokine production in the host, and that this response varied according to the length of storage. A shorter storage time was associated with a mixed pro- and anti-inflammatory response, whereas a more prolonged storage time elicited an anti-inflammatory response (7). There are also some recent data suggesting that the preservative solution further modulates this response (8). The clinical effects of RBC transfusion on the immunologic responses of critically ill children remain to be determined. In summary, some evidence suggests that transfusion may cause immunosuppression, thereby increasing the risk of acquiring nosocomial infections. RBC transfusion can also reinforce SIRS, which can progress to MODS.
patient with SIRS may stimulate their inflammatory syndrome, which may result in the development of MODS (second-hit theory of MODS). Some data suggest that the inflammatory risks of RBC transfusion decrease significantly if the packed RBC units are prestorage-leukocyte depleted. However, an in vitro study showed that even leukoreduced RBC units induced cytokine production in the host, and that this response varied according to the length of storage. A shorter storage time was associated with a mixed pro- and anti-inflammatory response, whereas a more prolonged storage time elicited an anti-inflammatory response (7). There are also some recent data suggesting that the preservative solution further modulates this response (8). The clinical effects of RBC transfusion on the immunologic responses of critically ill children remain to be determined. In summary, some evidence suggests that transfusion may cause immunosuppression, thereby increasing the risk of acquiring nosocomial infections. RBC transfusion can also reinforce SIRS, which can progress to MODS.
Transfusion of Red Blood Cells: Application to the PICU
Practice Pattern

Current Evidence
Transfusion in Severe Anemia. Some data suggest that severe anemia may increase the risk of mortality in critically ill mammals and human beings. In healthy animals undergoing acute hemodilution, evidence of heart dysfunction appears once the Hb concentration drops below 3.3-4 g/dL. However, animals with 50%-80% coronary artery stenosis can show evidence of ischemic insult to the heart with an Hb concentration as high as 7-10 g/dL (12).
Two studies undertaken in patients who refused blood products for religious reasons suggest that very low Hb levels may increase mortality (13,14). After bloodless surgery, the risk of death increased steadily once the postoperative Hb concentration dropped below 5-6 g/dL in healthy patients. A higher mortality risk was involved if heart disease was present, and the odds of mortality increased when Hb concentration dropped below 10 g/dL.
Few studies assess the relationship between anemia and mortality in acutely ill children. One group followed 2433 anemic African children who were hospitalized; 20% received a transfusion (15). Children with Hb 4.7 g/dL and respiratory distress who had received a whole blood transfusion had a lower mortality rate than those who did not receive a blood transfusion. Another prospective cohort study of 1269 Kenyan children hospitalized for malaria showed that whole blood transfusion decreased mortality if anemia was severe (Hb level <4 g/dL) or if dyspnea was associated with an Hb level of <5 g/dL (16). These studies suggest that some benefit may be associated with keeping the Hb concentration of hospitalized children above 5 g/dL. This might also be true for critically ill children, although some of these African children had chronic anemia due to sickle cell disease or malaria, which could modify their response to acute anemia, and the transfusions were whole blood, not leukoreduced RBC units.
All of these data indicate that it is not the absolute Hb value that is the primary determinant of the risk of mortality, but instead it is the risk of inadequate oxygen delivery to meet demand. Since oxygen delivery is determined by both cardiac output and oxygen content and oxygen demand is governed by metabolic rate, the use of Hb alone to determine the risk of mortality is very imprecise.
Red Blood Cell Transfusion: Current Guidelines
In 1942, two anesthesiologists, Drs. Adams and Lundy, wrote: “When concentration of Hb is <8-10 g per 100 cubic centimeters of whole blood, it is wise to give a whole blood transfusion before operation.” Thereafter, the “10/30” rule (Hb of 10 g/dL or hematocrit of 30%) was standard practice for more than 50 years, without support from high-quality clinical data. This concept also persisted despite the change from whole blood to the use of components and significant increase in the storage duration of RBC units. With recent publication of prospective RCT data, evidence-based medicine recommendations are now possible in some populations. Clinical situations requiring RBC transfusions can be divided into five categories: hemorrhagic shock, unstable patients, stabilized patients, patients with cyanotic heart disease, and chronic anemia.
Hemorrhagic Shock. Hemorrhagic shock is a specific clinical situation where shock is due partly to hypovolemia, which decreases cardiac output, and hence DO2, and partly to RBC loss, which decreases HB and hence DO2. Despite the high risk of mortality for this condition, no RCT has yet evaluated the proper RBC transfusion threshold in such a population. Therefore, physicians usually rely on expert recommendations. Both the Pediatric Advanced Life Support (PALS) and the Advanced Trauma Life Support (ATLS) recommend initiating volume resuscitation with 40-60 mL/kg of crystalloid solutions (normal saline or Ringer-Lactate) before transfusing RBC units. These current guidelines are based on animal data and very small human studies and are becoming more controversial with recent large observational studies of massive transfusion for hemorrhagic shock. (Massive transfusion is discussed elsewhere in this chapter.)
A recent trial in 921 adults with acute upper gastrointestinal bleeding demonstrated that, compared with a liberal transfusion strategy (Hb <9.0 g/dL), a restrictive strategy (Hb <7.0 g/dL) significantly improved mortality at 45 days (17). The percentage of patients who received a transfusion of fresh frozen plasma (FFP) or platelets and the total amount of fluid administered were similar in the two groups. These patients were not all in hemorrhagic shock, although the mean volume of fluid resuscitation was 5 L. This study suggests that trials on transfusion thresholds in hemorrhagic shock are needed.
Unstable Patients. An unstable patient can be defined as a patient with physical examination or laboratory evidence of shock (oxygen debt). Signs of shock include prolonged capillary refill, oliguria, abnormal level of consciousness, increased lactate levels, and decreased SvO2 or StO2 (tissue oxygen saturation). While hypotension itself may not confer shock, it clearly increases the risk of it. It is reasonable to transfuse RBCs for a threshold >7g/dL in children who are unstable; however, no consensus defines the threshold. In addition, other methods of
improving oxygen content or cardiac output should also be considered. How the oxygen delivery and consumption relationship is altered should be dependent upon the method that has the optimal risk to benefit ratio for the individual patient.
improving oxygen content or cardiac output should also be considered. How the oxygen delivery and consumption relationship is altered should be dependent upon the method that has the optimal risk to benefit ratio for the individual patient.
In an adult randomized clinical trial involving septic patients in an emergency room setting a rapid (<6 hours) and aggressive therapy-driven protocol with the specific goal of decreasing mortality in adults with severe sepsis and septic shock was studied (18). RBC transfusions to maintain the hematocrit over 30% were given to patients in the experimental group if the O2 saturation in the central cava vein was lower than 70% after fluid challenge, vasopressors, and inotropes. Mortality was 46.5% in the standard treatment group (n = 133) versus 30.5% in the “early goal-directed therapy” group (n = 130, p = 0.009). Unfortunately, the isolated effect of RBC transfusions in these patients could not be evaluated. The applicability of this trial to children is debatable, but it is possible that the outcome of patients with severe sepsis may be better if the Hb concentration is kept over 10 g/dL if all other aspects of the sepsis bundle employed in the study are maintained, at least during the first hours following diagnosis. The extrapolation of these results to other unstable patients remains uncertain.

In critically ill adults, the transfusion requirements in critical care (TRICC) trial showed that in hemodynamically stable, critically ill patients, a restrictive transfusion strategy (RBC transfusions with non-leukocyte-depleted units given only if the Hb concentration dropped under 7 g/dL and maintained at 7-9 g/dL) was safer than a liberal transfusion strategy (RBC transfusions given if the Hb concentration dropped under 10 g/dL and maintained at 10-12 g/dL) (19). An adjusted multiple-organ dysfunction score and the hospital mortality rate were statistically lower in the restrictive transfusion group.
In 2007, the pediatric transfusion requirements in PICU (TRIPICU) study randomized 320 stabilized critically ill children to a hemoglobin threshold of 7 g/dL for red cell transfusion (restrictive-strategy group) and 317 patients to a threshold of 9.5 g/dL (liberal-strategy group) (20). The number of patients who developed new or progressive MODS (primary outcome) was similar in both groups: 38 (11.9%) in the restrictive versus 39 (12.3%) in the liberal group. No differences were reported in other major outcomes, including all-cause mortality (14 deaths after 28 days in both groups), nosocomial infection, and length of stay. This noninferiority trial suggests that hemodynamically stable critically ill children have similar outcomes with less exposure to RBCs when a restrictive (<7 g/dL) versus a liberal (<9.5 g/dL) transfusion approach is used. These results were consistent in subgroup analysis performed in noncyanotic cardiac surgery patients, general surgery patients, and septic patients. These subgroup analyses were, however, underpowered and need to be confirmed in larger trials.

Chronic Anemia. Patients with chronic anemia (i.e., anemia that is not the result of acute blood loss nor acute hemodilution) have usually increased their cardiac output (to maintain DO2) or have decreased their [V with dot above]O2. No RCT has evaluated the transfusion threshold for these patients. The only data are from observational studies. In 61 adult Jehovah’s Witnesses with an Hb <8 g/dL, deaths due to anemia increased only at an Hb
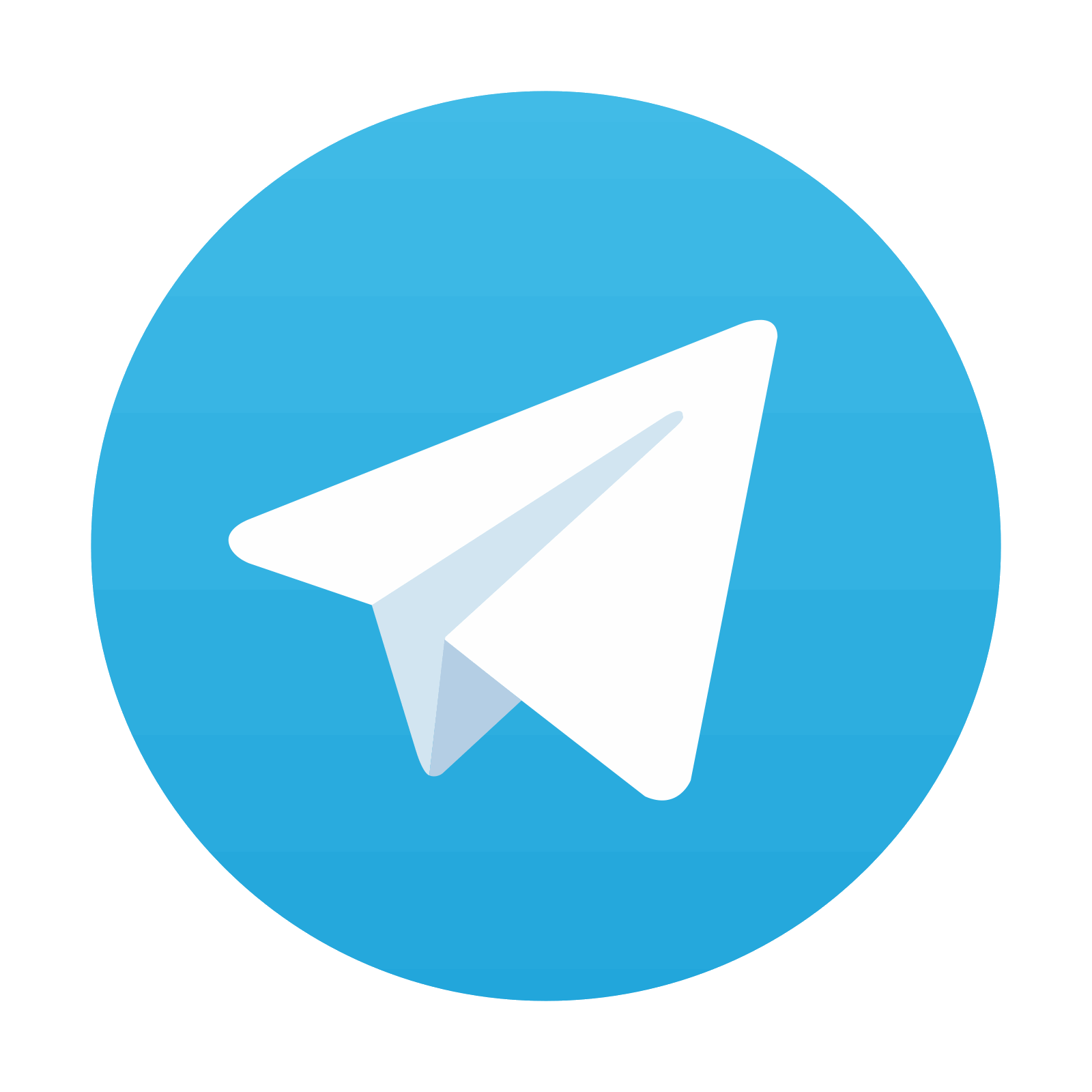
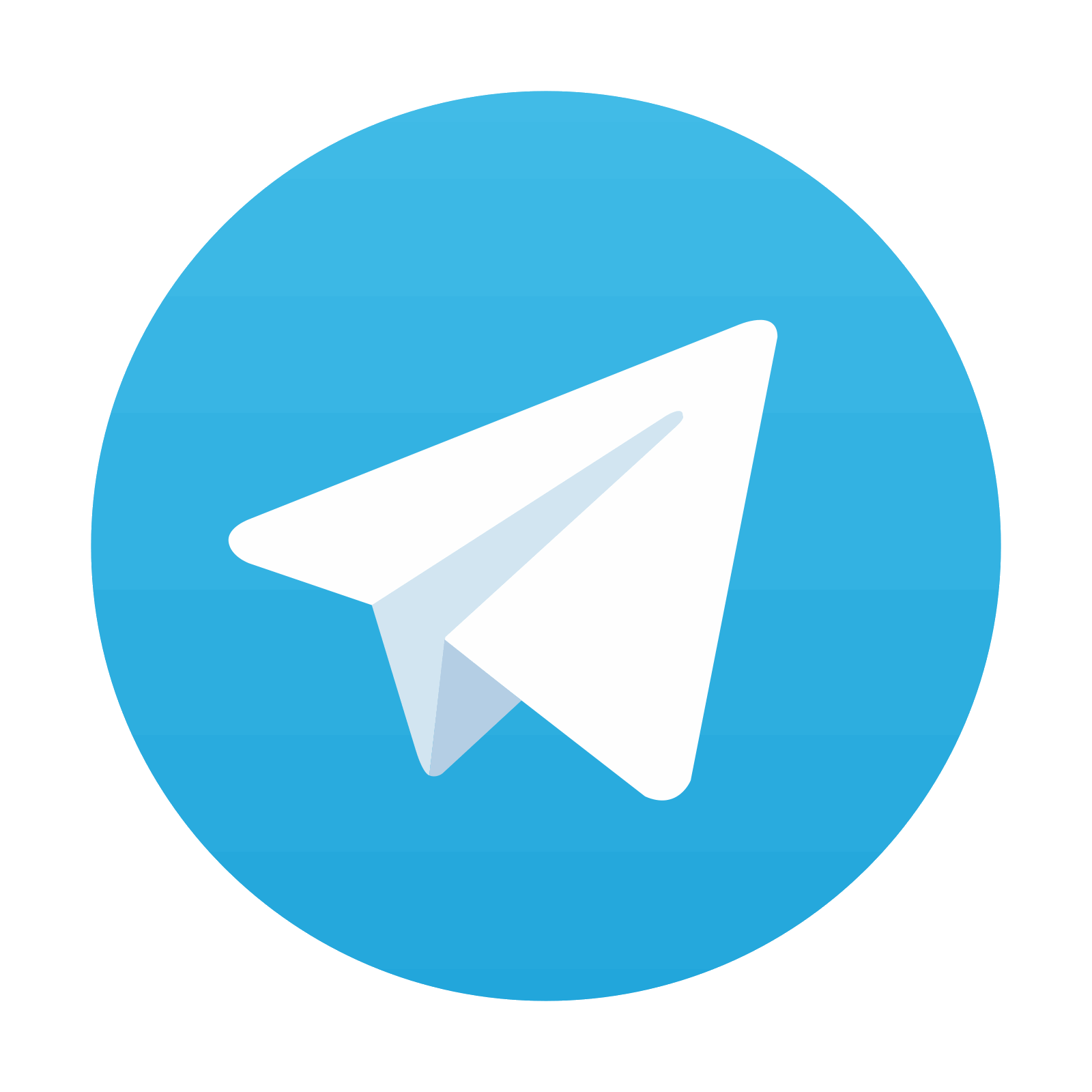
Stay updated, free articles. Join our Telegram channel
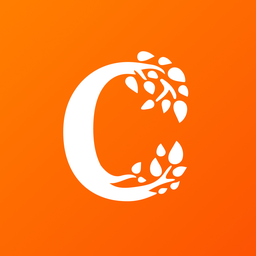
Full access? Get Clinical Tree
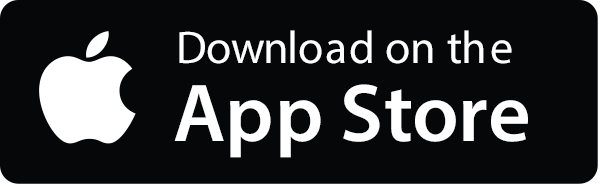
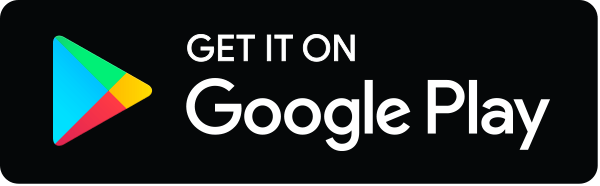
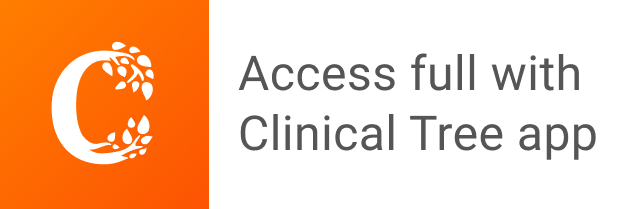