INTRODUCTION
Blood gases provide important clinical information for patients with respiratory disorders, compromised circulation, or abnormal metabolism. In recent years, a number of surrogates for blood gas analysis have entered the daily practice of emergency medicine. Leveraging the benefits of blood gases requires an understanding of the underlying physiology, appropriate use of arterial and venous blood gases, and knowledge of the advantages and limitations of noninvasive monitoring methods. This chapter will be limited to evaluation of oxygen and carbon dioxide levels; for information on carbon monoxide, please refer to chapter 222, “Carbon Monoxide.”
RESPIRATORY PHYSIOLOGY
Several factors contribute to overall gas exchange in the lungs. Each breath (tidal volume) is composed of functional movement of air in and out of the alveolus and nonfunctional movement of air through bronchioles, bronchi, trachea, and nonperfused areas of lung (physiologic dead space). The physiologic dead space is approximately 30% of the tidal volume. The air remaining in the chest at the end of exhalation is referred to as the functional residual capacity. Dead space and the functional residual capacity do not contribute to gas exchange. The amount of air inhaled and exhaled in a minute is referred to as minute ventilation and is the product of the respiratory rate and tidal volume. Relatively small changes in the functional alveolar space demand large increases in the minute ventilation to maintain the same rate of gas exchange. Either raising the fraction of inspired oxygen (Fio2) or increasing the surface area or functional residual capacity of the lung can increase total alveolar oxygen (O2) content. Positive-pressure ventilation increases the functional residual capacity through recruitment of collapsed nonventilated alveolar space.
The Fio2 is the fraction or percentage of oxygen in the space being measured. At sea level, room air is 21% oxygen (20% is often used for ease of calculation). As Fio2 increases, the alveolar concentration of oxygen (Pao2) proportionately increases. Unless the patient is part of a closed system, like a ventilator circuit, the Fio2 is only estimated. Each liter per minute of O2 flow delivered via nasal cannula increases the Fio2 by about 4%. Flow rates >4 L/min through a nasal cannula are poorly tolerated because of upper airway irritation. A simple O2 mask provides an Fio2 of 35% to 60% at flows of 10 to 15 L/min. A nonrebreather mask with a reservoir can deliver 95% O2 at a flow rate of 10 to 12 L/min.
Comparing the measured arterial concentration of oxygen to the expected concentration of oxygen can be useful for determining the nature and severity of respiratory disorders. The approximate arterial oxygen concentration (Pao2) values that are expected in normal persons who are inhaling various concentrations of O2 are listed in Table 16-1.
The expected Pao2 for a patient being given supplemental oxygen can be roughly estimated by multiplying the actual delivered percentage of O2 by 5, the “Five Times Rule.” Thus, a patient getting 60% O2 would be expected to have a Pao2 of about 60 × 5, or 300 mm Hg. For every 1000 ft (305 m) rise in altitude, barometric pressure drops approximately 25 mm Hg, and the atmospheric partial pressure of oxygen (Po2) drops about 5 mm Hg. The arterial oxygen estimate would therefore be reduced by the fraction of pressure at elevation compared to sea level. The total alveolar oxygen pressure cannot be greater than atmospheric pressure unless the patient is receiving positive-pressure ventilation. Further discussion of O2 and ventilation at altitude and depth is found in chapters 221, “High-Altitude Disorders” and 214, “Diving Disorders.”
Once oxygen or carbon dioxide reaches the alveolus, it moves across the interstitial space to either the red blood cell or alveolar space, respectively. The efficiency of diffusion depends on the distance across the alveolar-capillary membrane (interstitial space), the partial pressure of the gas in the alveolar space, and the solubility of the gas. Both oxygen and carbon dioxide are highly soluble, and carbon dioxide is 20 times more soluble than oxygen. As a result, increases in the distance across the interstitial space (as in pulmonary edema, for example) have greater effect on oxygen diffusion than carbon dioxide (CO2) diffusion. Gas diffusion requires functioning alveolar space, and conditions that damage the alveolus prevent effective oxygen and carbon dioxide transport. Additionally, the alveolar space must be perfused by the pulmonary circulation. When portions of lung are perfused but not ventilated (as in pneumonia), or ventilated but not perfused (as in pulmonary embolism), there is a ventilation-perfusion mismatch. Either scenario may lead to hypoxemia, the first as deoxygenated blood passes through the nonfunctional lung and mixes with oxygenated blood in the left atrium, and the second when too little perfused lung is available for adequate oxygen loading.
The effectiveness of alveolar oxygenation can be estimated either by the alveolar-arterial gradient or the Pao2/Fio2 ratio. The alveolar-arterial gradient is the difference between the partial pressure of oxygen in the alveolar space (estimated from Fio2 at atmospheric pressure) and the measured partial pressure of the gas in an arterial blood gas sample. The alveolar-arterial (A-a) gradient can be estimated by the following simplified formula:
A normal gradient for young adults is <15 mm Hg. The gradient increases with age at a rate of approximately 4 to 8 mm Hg per decade or can be estimated with the following formula: age/4 + 4. The Pao2/Fio2 ratio correlates with the relative venous shunt across the pulmonary circulation and is calculated as the measured Pao2 divided by the Fio2 in decimal form. A healthy person on 40% oxygen would be expected to have a ratio of approximately 600, representing a normal physiologic shunt of approximately 5%. As the shunt increases, the ratio decreases. Table 16-2 illustrates the fall in the Pao2/Fio2 ratio with increasing physiologic shunt in hypothetical patients all receiving oxygen with an Fio2 of 40%.
The Pao2/Fio2 ratio is the most frequently used parameter for evaluating the severity of lung failure and is included in the current definition for acute lung injury/acute respiratory distress syndrome.1 The amount of oxygen transported to the body is determined to the greatest extent by the total blood hemoglobin content and cardiac output. Relatively little oxygen is dissolved in the plasma itself. The arterial O2 content of blood (Cao2) can be calculated with the following formula (hemoglobin [Hb] in grams/dL, Pao2 in mm Hg, and arterial oxygen saturation [Sao2] expressed as a fraction of 1.0):
As a result, systemic delivery of oxygen can only be practically modified by either increasing the hemoglobin content or oxygen saturation. Although increasing the hemoglobin by transfusion will increase O2 content, various qualities of transfused blood limit its benefit to patients, and further discussion is beyond the scope of this chapter (see chapters 13, “Fluid and Blood Resuscitation in Traumatic Shock,” and 238, “Transfusion Therapy”).
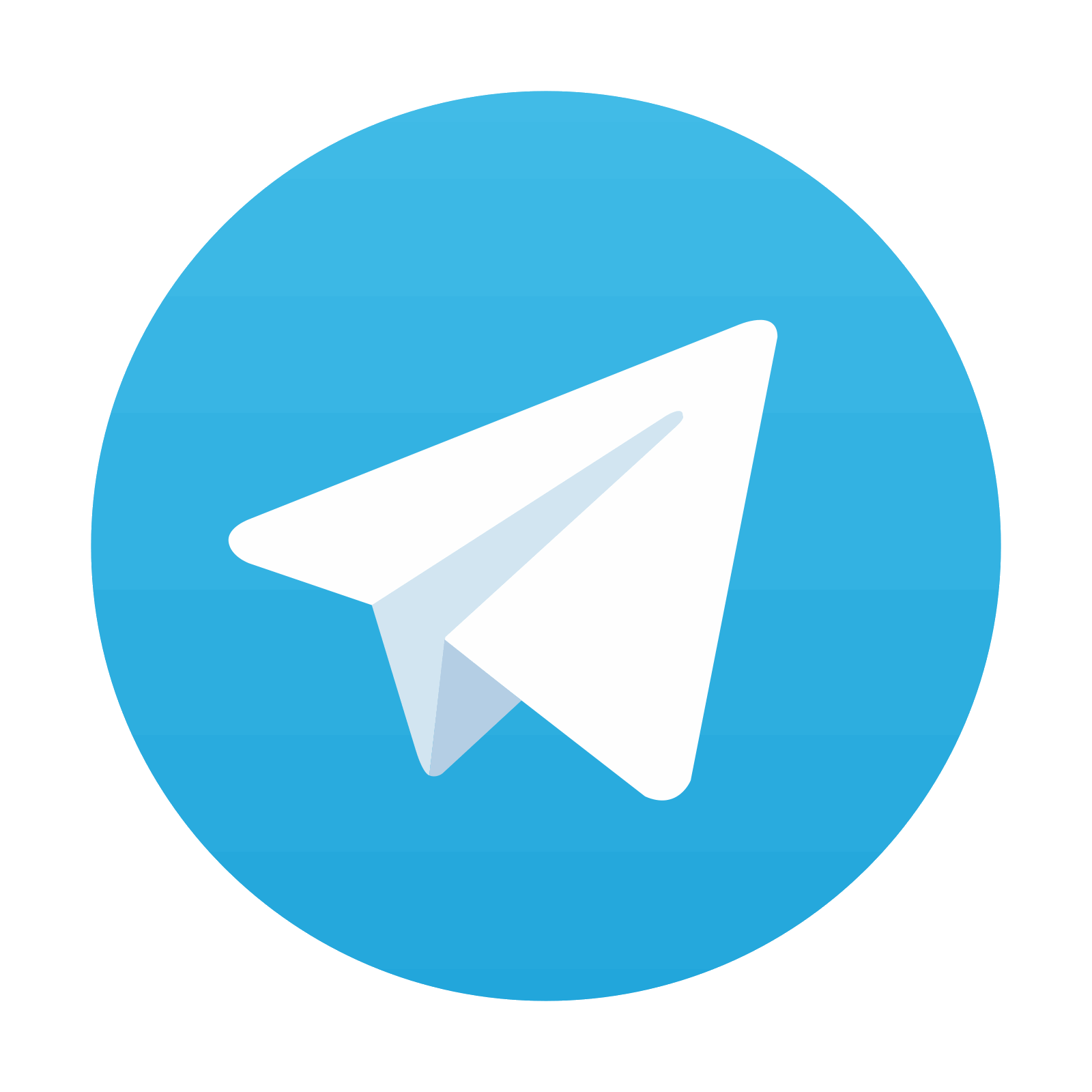
Stay updated, free articles. Join our Telegram channel
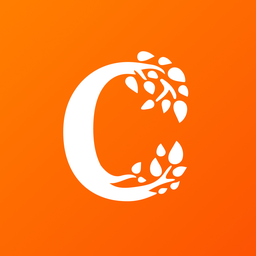
Full access? Get Clinical Tree
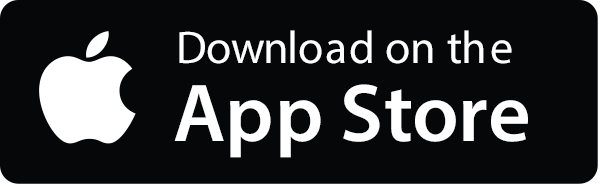
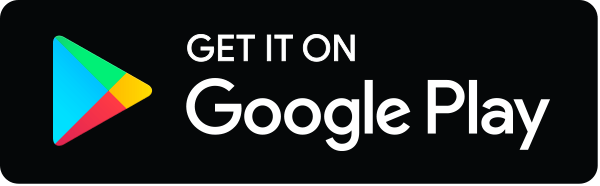