CHAPTER 14 Blood Conservation
Jean-Baptiste Denis is thought to be the first to attempt an intravenous transfusion of whole blood. He was a Parisian physician and astrologer who, in 1667, gave a teenage boy the blood of either a lamb or a dog in an attempt to restore him after he had been bled multiple times for fever. Denis was later tried for murder when another of his patients died. He was exonerated, but transfusion was subsequently banned throughout Europe for more than 100 years (Moore, 2003).
More than 300 years after the death of Pope Innocent VIII, the first successful human transfusion was performed in Philadelphia and credited to the University of Edinburgh-trained “Father of American Surgery,” Philip Syng Physick (Fig. 14-1). He did not publish any writings about his accomplishment and few details exist of the circumstances or outcome (Jepson, 1974).
Hemoglobin structure and function in the neonate, infant, and child
Hemoglobin, the primary oxygen-carrying pigment, is a large complex tetrameric protein consisting of iron-containing heme groups (protoporphyrin IX ring with attached ferrous iron atom) and the globin protein moiety (Fig. 14-2). The paired arrangement of polypeptide globin chains each interacting with an attached heme group provides the complex reversible interactions that allow for the transport of oxygen. Because each heme moiety has the capacity to bind a single oxygen molecule, a molecule of hemoglobin can transport as many as four oxygen molecules; remarkably, this process is accomplished without the input of energy.
Hemoglobin structure during the embryologic period is characterized by three hemoglobin species, which include Gower-1(ζ2ε2), Gower-2 (α2ε2), and Portland (ζ2γ2) (Fig. 14-3). By the tenth week of gestation, these embryonic hemoglobin species are nearly completely replaced by fetal hemoglobin (α2γ2), which is also called hemoglobin F. At 10 to 12 weeks of gestation, the distribution of hemoglobin is about 80% to 90% fetal hemoglobin and 10% hemoglobin A. Synthesis of fetal hemoglobin ceases at approximately 38 weeks of gestation; at birth, the percentage of fetal hemoglobin has decreased to about 70% to 80% and, under normal circumstances, continues to decrease thereafter. By 6 months of age, fetal hemoglobin levels typically have decreased to less than 5% and by 1 year of age, to 2%, which is a level similar to that in adults.
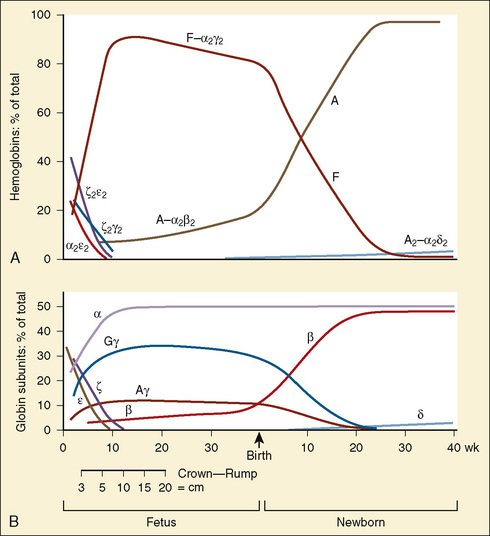
(Modified from Polin RA, Fox WW: Fetal and neonatal physiology, ed 2, Philadelphia, 1998, Saunders.)
The primary physiologic function of hemoglobin is to carry oxygen acquired in the capillary beds of the pulmonary alveoli (or, in fetal life, the chorionic villi of the maternal placenta) and release it in the reduced-oxygen environment of the tissues. Hemoglobin also is important as a biological buffer and in the transport of both carbon dioxide (the Bohr effect) and nitric oxide (Fig. 14-4). In its primary role as an oxygen carrier, hemoglobin functions by altering its affinity for oxygen through changes in the quaternary structure of the protein moiety. This relationship can be usefully illustrated by examining the appearance of the oxyhemoglobin dissociation curve (Fig. 14-5). The sigmoidal shape of the curve, which describes the relationship of hemoglobin saturation at various levels of oxygen tension, results from the four globin chains individually interacting with oxygen and collectively affecting the affinity of the other chains for oxygen. This complex interaction results in an affinity curve that demonstrates hemoglobin’s low affinity (flat portion of the lower curve) for oxygen in hypoxic environments and a rapidly increasing affinity (steep portion) as oxygenation of each heme group occurs until the molecule becomes saturated (flat portion of upper curve). The classic shape of the curve is described for hemoglobin A and reflects the physiologic requirement to load and unload oxygen within a narrow range of oxygen tensions. Other hemoglobin species and mixtures of species have different affinities for oxygen and therefore produce different dissociation curves that in turn reflect embryonic or fetal needs.
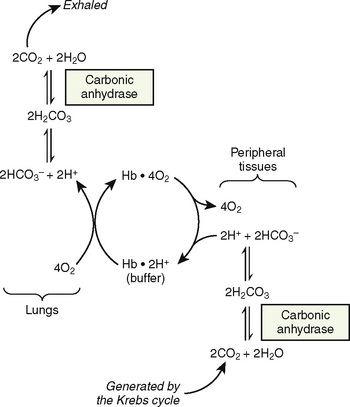
(Modified from Polin RA, Fox WW: Fetal and neonatal physiology, ed 2, Philadelphia, 1998, Saunders.)
The dissociation curve for fetal hemoglobin reflects its function as an acceptor of oxygen carried by maternal hemoglobin A. Therefore, fetal hemoglobin must have a greater affinity for oxygen than maternal hemoglobin (hemoglobin A) to accept oxygen carried to the uterine villi. The increased affinity of fetal hemoglobin for oxygen can be traced to its lower capacity to interact with 2,3-diphosphoglycerate (2,3-DPG), because the binding site for 2,3-DPG is on the β chain, which is absent in fetal hemoglobin (Jepson, 1974). Although one would expect that the increased affinity for oxygen characteristic of fetal hemoglobin would be essential for adequate oxygen delivery in the fetus, that appears not to be the case, as illustrated by the lack of deleterious effects on the fetus when hemoglobin A is transfused in utero (Mathers et al., 1970). Furthermore, infants born to mothers with hemoglobinopathies characterized by an increased affinity for oxygen show no apparent effects (Moore et al., 1967). By extension, it can be presumed that in the neonate, transfusion with blood containing hemoglobin A is not harmful and may in fact have clear advantages, especially in critical illness (Oski, 1973).
As previously discussed, the characteristic sigmoidal shape of the oxyhemoglobin dissociation curve of hemoglobin A is a reflection of its structure, whereas its position with respect to oxygen saturation and oxygen tension is a function of various factors. Under normal circumstances, the oxygen tension at which hemoglobin A is 50% saturated (P50) is 27 mm Hg. Temperature, pH, Pco2, and 2,3-DPG levels all have profound effects on oxygen affinity and therefore on P50, resulting in a leftward or rightward shift in the position of the curve. Because the appearance of the oxyhemoglobin dissociation curve for the neonate is identical to that of the adult at a pH of 7.6, environmental differences are potentially more important to hemoglobin’s functional affinity than are fundamental differences in the hemoglobin molecule itself (Nelson et al., 1964). Under normal circumstances, the P50 increases from about 19 mm Hg at 1 day of age to the adult level of 27 mm Hg at age 4 to 6 months (Fig. 14-6). At the end of the first year, the P50 actually exceeds that of the adult at a level slightly greater than 30 mm Hg (Oski, 1973). For further reading, see Chapter 3, Respiratory Physiology in Infants and Children.
Physiologic anemia and the anemia of prematurity
At term, the neonate has a hemoglobin concentration of approximately 17 g/dL. As hemoglobin F is replaced with hemoglobin A over the ensuing months, the hemoglobin level decreases to a low of 10 g/dL, and the oxyhemoglobin dissociation curve shifts rightward. This shift is the result of the combination of increasing levels of hemoglobin A and increased levels of 2,3-DPG as described by Oski (1973). An increase in the levels of either one alone is insufficient. The importance of this is apparent in infants who have respiratory distress syndrome with abnormally low levels of 2,3-DPG and who show improved oxygen unloading at the tissue level after transfusion with fresh adult blood. The transition from a P50 of 19 mm Hg in the term neonate to 27 mm Hg (as in the adult) typically occurs over 4 to 6 months. However, in the premature patient this transition may be delayed to as late as age 12 months.
At birth, oxygen tensions increase quickly, effectively halting erythropoietin synthesis, and consequently, erythropoiesis. In the full-term neonate, erythropoietin and, consequently, hemoglobin levels begin increasing around the age of 4 months, resulting in the correction of physiologic anemia, which is often called the physiologic nadir to emphasize the physiologic or nonpathologic nature of the decrease in hemoglobin. In premature infants, the phenomenon is more complex and is complicated by the need for repeated blood sampling in hospitalized premature infants. Often, the blood sampling requirements equal or exceed half of the total blood volume in infants weighing less than 1 kg (Stockman, 1986). The need for phlebotomy results in a need for the transfusion of adult banked blood—blood containing hemoglobin A with its characteristic lower affinity for oxygen. The resulting increase in tissue-oxygen tension further decreases erythropoietin synthesis and prolongs the duration of anemia for a period that is dependent on factors such as weight, gestational age, and the ongoing need for transfusion.
The anemia of prematurity is a true anemia that produces clinical signs and symptoms such as tachycardia, bradycardia, apnea, delayed growth, and poor weight gain. Treatment is directed at these consequences and consists of either transfusion, or more recently, the use of recombinant erythropoietin. Studies examining the potential benefit of booster transfusions targeted to keep hemoglobin levels greater than 10 g/dL in premature infants have been mixed; some have shown improvements in weight gain, and others have failed to demonstrate benefit (Blank et al., 1984; Stockman et al., 1984; Keyes et al., 1989).
The use of recombinant erythropoietin has also been studied extensively in the treatment of anemia of prematurity. A Cochrane Review described the results of 23 studies involving 2074 infants (Fig. 14-7) (Ohlsson and Aher, 2006). The end point of the majority of the studies was a reduction in the need for transfusion and donor exposure after enrollment. In the majority of studies, the need for transfusion was reduced; however, the reduction is of limited clinical significance. Several studies also found an increased incidence of retinopathy of prematurity among those treated with erythropoietin. The lack of clinically relevant benefit and the potential to increase the occurrence of retinopathy of prematurity prompted the recommendation against the use of erythropoietin as a means of reducing the need for transfusion in premature infants. Transfusion practices and indications in this group are discussed later in this chapter.
Perioperative strategies for blood conservation
Erythropoietin
As discussed in the previous section, erythropoietin is an inducible glycoprotein produced in the kidneys and extrarenal tissues. It regulates erythropoiesis in response to tissue hypoxia. Acute anemia is associated with exponential increases in erythropoietin in the plasma. However, in the critically ill, erythropoietin induction is blunted, as has also been observed in various chronic illnesses in childhood. The exact mechanism of the inhibition observed in both critical illness and chronic illness has not been described (Krafte-Jacobs et al., 1994). Regardless of the mechanism, both situations often lead to the need for blood transfusion. The use of recombinant erythropoietin has been investigated as a means of reducing the need for transfusion or the frequency of transfusion in chronic or critical illness.
Adult studies have shown mixed results in the efficacy of recombinant human erythropoietin to avoid or limit blood transfusion. Several randomized controlled studies, including a study reported by Silver et al. (2006), have shown that at least 1 unit of blood was saved in patients in an adult intensive care unit (Corwin et al., 2002). More recently, however, Corwin and colleagues (2007) reported that in a large, prospective, randomized controlled trial involving more than 1400 adult patients, the group receiving erythropoietin had a 10% decrease in the need for transfusion compared with the control group. However, that study and others found an increase in the instances of thrombotic events among patients receiving erythropoietin.
A benefit, albeit small, was found in studies that examined the use of perioperative erythropoietin for reducing the need for transfusion during and after procedures in which transfusion is normally required. Laupacis and Fergusson (1998), in a meta-analysis involving adults undergoing either orthopedic or cardiac surgery, found a significant reduction in allogenic RBC transfusion in both groups of patients (Fig. 14-8). In a study of children undergoing craniofacial repair, Helfaer et al. (1998) found that children receiving preoperative erythropoietin required transfusion significantly less often than controls (64% vs. 100%). Other small studies (183) and case reports have concluded that erythropoietin is efficacious in reducing the need for RBC transfusion in children, but in the absence of data from large, well-designed prospective trials, its use cannot be recommended.
Iron Supplementation
The incidence of iron deficiency anemia is approximately 9% among children 1 to 2 years old in the United States (Looker et al., 1997). For adult patients with normal iron storage, there is conflicting evidence as to whether iron supplementation perioperatively improves the hemoglobin level. Several randomized control trials have failed to show that oral iron supplementation increases hemoglobin levels perioperatively (Crosby et al., 1994; Sutton et al., 2004; Mundy et al., 2005). However, two clinical trials (one randomized and one nonrandomized) with colorectal surgical patients have shown that treatment with oral iron supplementation for two weeks significantly increased hemoglobin levels and decreased blood transfusion rates (Okuyama et al., 2005; Lidder et al., 2007). The conclusion of a review by Beris et al. (2008) for the Network for Advancement of Transfusion Alternatives was that there is insufficient evidence to recommend the use of intravenous iron as a means of reducing the need for perioperative transfusion in adults. As with the use of erythropoietin, few data for children are available.
Hemostatic Drugs
Three currently available hemostatic drugs have been well investigated and extensively used to limit blood loss perioperatively. Two of the drugs, aminocaproic acid (EACA) and tranexamic acid (TA), are lysine amino-acid synthetic derivatives; the third, aprotinin, is a naturally occurring antifibrinolytic and proteinase inhibitor (Fig. 14-9). These drugs have been extensively used in adults and, more recently, in children.
Fibrinolysis, the lysis of formed fibrin clot, results from the enzymatic conversion of the proenzyme plasminogen to plasmin, a process that is mediated by tissue plasminogen activator, urokinase, factors XIa and XIIa, and kallikrein. Fibrinolysis results in the cleavage of polymerized fibrin strands at multiple sites and releases fibrin degradation products such as D dimer (Kolev and Machovich, 2003). EACA and TA exert their antifibrinolytic activity by reversibly blocking the lysine binding site on plasminogen, preventing binding to fibrin and conversion to active plasmin. As an inhibitor of fibrinolysis, TA is 10 times more potent than EACA. TA may also improve hemostasis by preventing plasmin-induced platelet activation, and both EACA and TA have antiinflammatory properties, but they are less than those of aprotinin (Eaton, 2008).
Antifibrinolytics have been used in children primarily for spine surgery and cardiac surgery, although they have been used for other procedures, including craniofacial reconstruction and repair of congenital diaphragmatic hernia during extracorporeal membrane oxygenation (ECMO). Sethna et al. (2005) reported on a randomized study of 44 pediatric patients undergoing elective spine surgery who received either TA (100 mg/kg loading dose followed by 10 mg/kg per hour) or saline placebo during the procedure; the treatment group had a 41% reduction in blood loss (Fig. 14-10). In a subsequent study involving children with Duchenne’s muscular dystrophy, the same authors found a similar reduction in the transfusion requirement (Shapiro et al., 2007).
For adult cardiac surgery, the use of antifibrinolytics is well established. For children, although the efficacy data are less available and of lower quality, they support the use of TA and EACA primarily in children undergoing repair of cyanotic congenital heart disease. Bulutcu et al. (2005), in a series of studies involving 750 cyanotic patients, found that both TA and EACA were beneficial in reducing transfusion requirements by up to 50%, reducing blood loss by 44%, and significantly reducing times for sternal closure and rate of reexploration.
Few complications have been associated with the use of TA and EACA, although concerns have been related to thrombosis in patients, such as those undergoing ECMO or a Fontan procedure requiring the use of a baffle fenestration. Although case reports suggest the potential for concern, studies involving 71 patients undergoing a Fontan procedure and 431 patients undergoing ECMO have failed to demonstrate an increased risk of thrombosis (Hocker and Saving, 1995; Gruber et al., 2000; Downard et al., 2003).
Aprotinin is a nonspecific serine protease inhibitor derived from bovine lung that inhibits proteases with active serine residues, especially plasmin. The resulting effects are an attenuation of inflammatory responses and antifibrinolysis. Aprotinin and the lysine analogues have very different modes and scopes of action, but ultimately both function by inhibiting fibrinolysis through the inhibition of plasmin. Additionally, aprotinin is thought to restore the adhesive properties of platelets independently of its effect on the inhibition of fibrinolysis (Bradfield and Bode, 2003). The efficacy of aprotinin is somewhat less clear than that of either EACA or TA. Eaton (2008), in a comprehensive review published in 2008, described in detail the available data on the efficacy of aprotinin use in pediatric cardiac surgery, in which it is most often used. Of the 14 randomized controlled trials, 11 showed a reduction in at least one parameter of blood loss or replacement. Three studies by Boldt et al. (1994, 1993a, 1993b) showed no benefit, whereas the majority of observational studies described in Eaton’s review did show benefit. The difficulty in evaluating the extensive literature on the use of aprotinin in pediatric cardiac surgery lies in the differences in end points (e.g., transfusion requirement, or chest tube output), dosing regimens (i.e., high dose vs. low dose), surgical procedures (e.g., Fontan procedure or ventricular septal defect), cardiopulmonary bypass management, and patients (e.g., infants, neonates, or reoperation). The relatively small numbers evaluated within each study and the virtually endless potential permutations of the resulting data make drawing conclusions about efficacy difficult, although the weight of the existing evidence in adults and children suggests a benefit similar to that obtained with the lysine derivatives TA and EACA.
The use of aprotinin has raised concerns about the potential for complications, including thrombosis, anaphylaxis, and, most importantly, renal failure. In 2006 the Multicenter Study of Perioperative Ischemia Research Group reported on the largest observational prospective study of antifibrinolytic therapy (Mangano et al., 2006). The study tracked 4374 patients undergoing coronary artery bypass grafting and compared the use of aprotinin (1295 patients), EACA (883 patients), and TA (822 patients) with placebo (1374 patients). Aprotinin was associated with higher risks of death, cardiovascular event, cerebrovascular event, and renal failure. EACA and TA were not associated with increases in renal, cardiac, or neurologic complications. All three agents decreased blood loss to essentially the same degree.
In 1993, the Food and Drug Administration (FDA) approved aprotinin for patients at high risk of bleeding who were undergoing coronary artery bypass grafting with cardiopulmonary bypass (Ray and Stein, 2008). After the publication of the Blood Conservation Using Antifibrinolytics in a Randomized Trial (BART) study (Fergusson et al., 2008), Bayer Pharmaceuticals notified the FDA of its intent to withdraw aprotinin from the market. In that study of 2331 high-risk adult cardiac surgery patients, the investigators sought to determine whether aprotinin was superior to either TA or EACA in decreasing significant postoperative bleeding. The trial was terminated early because of an excess of deaths in the aprotinin group (6%) compared with the TA group (3.9%) and the EACA group (4.0%) (Fig. 14-11).
Preoperative Autologous Blood Donation
Since the 1980s, preoperative autologous donation (PAD) of blood 2 to 3 weeks before the operation has been used for adult cardiac and noncardiac surgical procedures in which blood loss and the need for blood transfusion are expected. The primary goal is to decrease the amount of allogenic blood transfused (Nath and Pogrel, 2005; Schved, 2005; Ferraris et al., 2007).
Numerous studies have documented the safety and benefit of this practice for adults in various settings. The main benefit is that it decreases the exposure to allogenic blood. A concern, though, is the amount of blood transfused (both allogenic and autologous) in patients who undergo PAD (Henry et al., 2002). The increased rate of transfusion is thought to lead to an increased risk of administrative errors with the increased number of units transfused (Schved, 2005). Vega et al. (2008) reported statistically higher complication rates among patients having reconstructive breast surgery who had PAD compared with patients in a control group who did not preoperatively donate their own blood.
Masuda et al. (2000) studied children weighing less than 20 kg. The children were not given erythropoietin, and each child predonated a mean (with standard deviation indicated as SD) of 48 (SD = 17) mL/kg of blood over an average of 50 (SD = 16) days. No child in the study group received allogenic blood transfusion, but 80% of children in the control group did. Sonzogni et al. (2001) pretreated children in a PAD group with subcutaneous erythropoietin 3 times a week for 3 weeks preceding cardiac surgery and once intravenously on the day of the operation. The controls were 39 consecutive age-matched patients from the previous year. Children predonated 9 mL/kg of blood on two separate occasions if the hematocrit was greater than 33%. Three of the 39 children in the study group required transfusion with allogenic blood, compared with 24 of the 39 in the control group.
Most studies of PAD in orthopedics involve scoliosis surgery. Murray et al. (1997) studied 243 consecutive pediatric patients undergoing spinal fusion and found that 90% of the children who predonated did not require allogenic blood during surgery. Moran et al. (1995) reported similar results in their study of children undergoing spinal fusion. In that study, the proportion of patients who needed allogenic blood (11%) was nearly identical to that found by Murray and colleagues. In both studies, at least 70% of the children were able to complete the donation process. Concern about the ability of children, especially young children, to complete the donation program is often cited as an obstacle to PAD. However, in both of these studies, children younger than 10 years successfully completed the donation process. Clearly, the ability of infants, toddlers, and young school-aged children to tolerate the donation process is uncertain at best. The use of sedation or general anesthesia to facilitate this process would seem to be somewhat unreasonable and excessive, although it has been reported (Velardi et al., 1998). A contraindication for PAD includes predonation anemia.
Acute Normovolemic Hemodilution
ANH is a technique designed to decrease the need for allogenic blood transfusion by the preoperative withdrawal of the patient’s own blood to be reinfused as needed during the subsequent procedure. The withdrawn blood is collected in standard blood-collection bags containing anticoagulant. The circulating volume is restored with either colloid (1:1) or crystalloid (3:1), thereby reducing the hematocrit and the potential for loss of RBC mass as bleeding occurs during the procedure (Helm et al., 1996; Ferraris et al., 2007). Children weighing less than 35 kg typically do not fill an entire collection bag. As bags typically contain approximately 63 mL citrate-phosphate-dextrose (CPD) and hold 450 mL blood, the volume of the anticoagulant needs to be reduced if the volume of collected blood is less than 450 mL. Studies of adults have examined the potential benefits of various replacement fluids, including hetastarch, albumin, and Lactated Ringer’s solution. All appear to be acceptable in adults, but few data exist to guide practice in children. Given that phlebotomy takes place just before the procedure and the withdrawn blood never leaves the patient’s operating room, the potential for error in transfusing the wrong blood to the wrong patient is greatly reduced. The withdrawn fresh whole blood, which contains the non-RBC components not found in banked blood, is reinfused at the end of the procedure.
The volume of blood to be removed can be calculated with the following formula:
where V is volume to be removed; EBV is estimated blood volume; Hi is initial or starting hematocrit value; Hf is the final or desired hematocrit value; and Hav is the average hematocrit value. The final or desired hematocrit is unclear and is dependent on various factors, including age, procedure, coexisting disease, and expected blood loss. Some have suggested that young infants are not appropriate candidates for ANH, because they have a limited capacity to increase cardiac output in response to anemia and the presence of fetal hemoglobin may limit oxygen unloading (Weldon, 2005). Supporting data, however, are lacking. In studies of older children and adolescents, hematocrit values have been allowed to decrease to as low as 9% without the development of lactic acidosis or other evidence of hypoperfusion (Fontana et al., 1995). The lowest appropriate or safe level of the desired hematocrit value is a function of the critical hemoglobin, which is the hemoglobin or hematocrit value below which oxygen consumption becomes delivery dependent and the ability to increase cardiac output is exceeded as lactic acidosis develops, reflecting insufficient oxygen delivery to the tissues. The critical hemoglobin concentration for humans is unknown, although studies in young healthy volunteers have failed to produce evidence of insufficient oxygen delivery at hemoglobin concentrations as low as 4.8 g/dL despite pharmacologic maneuvers that limited oxygen delivery to 7.3 mL/kg per minute (Lieberman et al., 2000). With the absence of cardiovascular disease in children, the ability to tolerate hematocrits of this level can be reasonably assumed; however, no large body of data exists to support the safety of the practice in children. Safety concerns must be balanced against the evidence for benefit usually determined by a reduction in total allogenic blood transfused or the reduction in exposure to units of allogenic blood. Linderkamp et al. (1992) calculated the following minimally acceptable hemoglobin concentrations: 6 g/dL for children and adults, 12 g/dL for preterm infants at birth, and 11 g/dL for full-term neonates at birth. These critical values were determined from oxygen transport parameters and oxygen consumptions (Linderkamp et al., 1992). Of course, the size of the preterm infant and the full-term neonate would preclude them from ANH; sufficient blood could not be drawn without risking a suboptimal hemoglobin concentration.
Several prospective randomized studies in adults show a modest decrease in the need for allogenic blood transfusion in both cardiac and noncardiac surgery (Goodnough et al., 1994; Moran et al., 1995; Kumar et al., 2002). In a 1998 meta-analysis, Bryson et al. did not demonstrate clear benefit with regard to a reduction in exposure to allogenic blood. According to the Society of Thoracic Surgeons Practice Guidelines, in the adult population the usefulness of ANH is not well established but may have benefit when used in conjunction with other blood conservation strategies (Masuda et al., 2000; Ferraris et al., 2007). A small series of adult patients of the Jehovah’s Witness faith who underwent live donor hepatic transplants demonstrated a clear benefit when their results were compared with those of historical controls (Jabbour et al., 2005). The patients in this series not only underwent ANH but also were treated preoperatively with erythropoietin to increase the preoperative level of hemoglobin. The potential benefits of ANH or a combined approach to blood conservation remain unclear and can be addressed only by well-designed studies of sufficient size to provide clinicians with reassurance that the benefit outweighs any potential risk.
Deliberate Hypotension
Controlled, deliberate, or induced hypotension is a method of blood-conservation strategy first described by Cushing in 1917. Since then, the technique has been applied to numerous procedures in various settings, with a multitude of different pharmacologic agents, and with patients of all ages. In a literature review, Tobias (2002) specifically looked at agents and techniques that can be used in infants and children.
Deliberate hypotension is defined as mean arterial pressure of 50 to 65 mm Hg, or 30% below baseline, which decreases bleeding in the surgical field and may be indicated for any procedure in which a relatively bloodless operating field is needed or whenever blood loss can be expected to require transfusion (Degoute, 2007). The ideal pharmacologic agent to induce controlled hypotension has the following characteristics: short onset, easily reversible and titratable, minimal toxic metabolites, minimal effects on vital organs, and predictable dose-dependent effect (Degoute, 2007). Numerous agents have been used successfully in both adults and children. A comprehensive review of agents useful in pediatrics was prepared by Degoute (2007). A summary of drugs is shown in Table 14-1.
The efficacy of deliberate hypotension to reduce the need for transfusion and improve the quality of the surgical field has been studied extensively for more than 30 years, although no large well-designed study or meta-analysis has demonstrated its efficacy in children. Still, the technique is widely accepted and used in children and infants. Most recent studies have focused on techniques and agents that may be used to achieve hypotension rather than on safety or efficacy. Shear and Tobias (2005), however, published a small study of cerebral oxygenation during controlled hypotension to 55 to 65 mm Hg using near infrared spectroscopy. They found that even with mean pressures of less than 54 mm Hg, oximetry values never decreased to less than 20% below baseline. They concluded that deliberate hypotension within the limits of 55 to 65 mm Hg was safe with regard to cerebral oxygenation.
Techniques for achieving deliberate hypotension are primarily pharmacologic, although positioning and regional techniques are occasionally used. The pharmacologic agents are derived from several different classes, including ganglionic blockers (e.g., trimethaphan), vasodilators (e.g., nitroglycerin and nitroprusside), β-blocking agents (e.g., esmolol), calcium channel blockers (most often nicardipine), and the volatile agents (presumably sevoflurane, with its greater titratability). The review by Tobias (2002) provides an extensive discussion of various agents. Often a combination of agents is required to provide adequate control of blood pressure, especially in young, healthy adolescents. The use of vasodilators such as nitroprusside or a volatile agent often results in reflex tachycardia that limits hypotension. Most practitioners find that satisfactory hypotension can be achieved only when heart rate is controlled, typically with a β-blocker (e.g., esmolol) or with a combination agent that blocks both α and β receptors (e.g., labetalol). Other agents that may be helpful in controlling heart rate, include clonidine (an α-receptor agonist) or a narcotic (especially remifentanil), as was shown in endoscopic sinus surgery and middle ear procedures (Degoute et al., 2003; Eberhart et al., 2003).
Dexmedetomidine has gained acceptance in various settings, but its use in this setting would appear to be limited. Nonetheless, it has been compared with remifentanil in two studies (one adult and one pediatric). In neither study was dexmedetomidine found to be superior (Tobias, 2002; Richa et al., 2008). A third article described only one patient for whom dexmedetomidine was used successfully to induce hypotension for spine instrumentation (Tobias and Berkenbosch, 2002). In the absence of additional data from larger prospective studies, the use of dexmedetomidine for controlled hypotension cannot be recommended.
Although the use of deliberate hypotension is widely accepted, the practitioner must be attentive to safety concerns, primarily those related to focal ischemia to such vital areas as the retina, spinal cord, and brain. Deliberate hypotension is contraindicated in patients with compromised circulation involving any critical vascular bed, elevated intracranial pressure, profound anemia or polycythemia, or sensitivity to any of the proposed hypotensive agents. The hematocrit concentration should be maintained at an adequate level. Despite the suggestion by some that deliberate hypotension and extreme ANH may be safely combined, deliberate hypotension should never be combined with ANH because of the risk of end organ ischemia (Schaller et al., 1983). The anesthesiologist must have experience with the technique and with the agents used to achieve hypotension. Adequate monitoring is essential, including invasive arterial blood pressure in all cases and central venous pressure monitoring in many or most cases. Patients placed in the reverse Trendelenburg position or in any position in which the head is higher than the heart must have the arterial pressure monitored and zeroed to reflect cerebral perfusion pressure. Arterial blood gases should be monitored frequently to ensure that a metabolic acidosis is not present, suggesting poor tissue perfusion or cyanide toxicity, when using sodium nitroprusside. Owing to its potential for toxicity, sodium nitroprusside deserves additional mention.
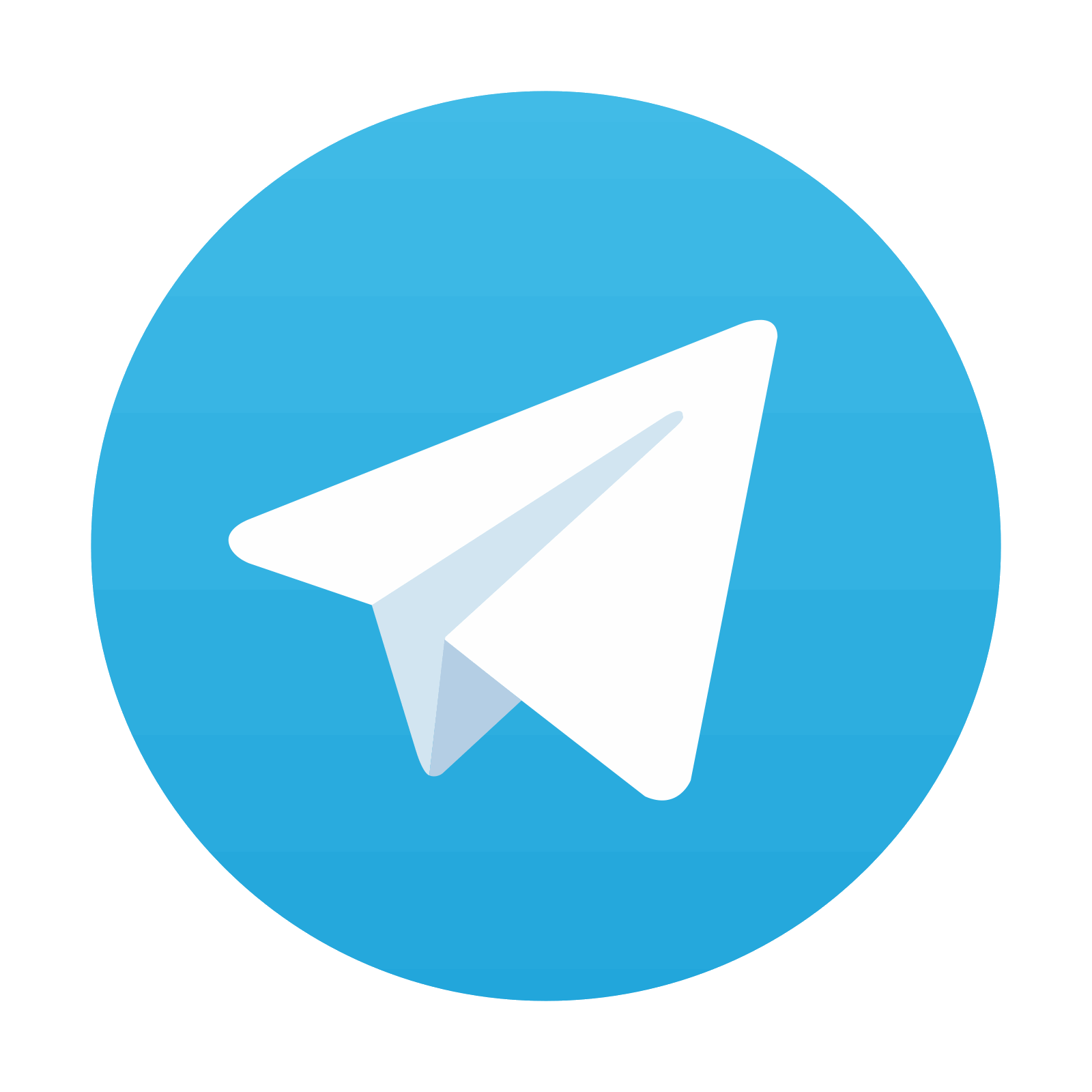
Stay updated, free articles. Join our Telegram channel
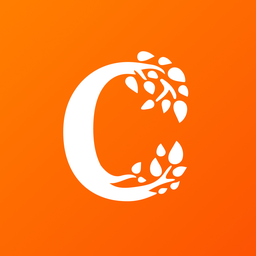
Full access? Get Clinical Tree
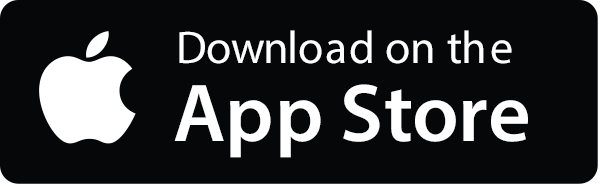
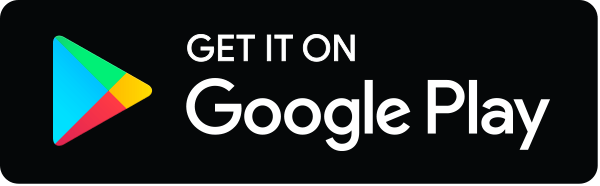