Fig. 10.1
Examples of jacketed projectiles and energies at muzzle velocity
10.1.1 Wound Ballistics and Penetrating Ballistic Injuries
The terminal effects of projectiles have been observed via field experience since the invention of firearms, and were initially investigated using post-mortem human subjects and animal models. Some of the first detailed investigations on wound ballistics were published in the 1800s, identifying the importance of projectile velocity on the resulting wound and the expansion of the tissue during an impact (Cooper and Dudley 1997). Today, the effects of projectiles are often investigated using tissue surrogate materials such as ballistic soap or ballistic gelatin (Sellier and Kneubuehl 1994) since they provide a higher degree of consistency and improved opportunities to observe the transient effects during an impact. One of the most widely used surrogates is ballistic gelatin, which is produced from biological materials (e.g. skin, bone and tendons) through extraction with hot water in an acidic (type A) or alkaline (type B) environment, and then combined with water, heated and mixed, and conditioned at specific temperatures for a period of 2–3 days before use (Jussila 2004). An impact between a steel sphere and 10 %, 4C gelatin (Fig. 10.2) progresses with the development of a temporary or expanded cavity, followed by maximum dynamic penetration and finally static penetration with the wound tract identifiable.
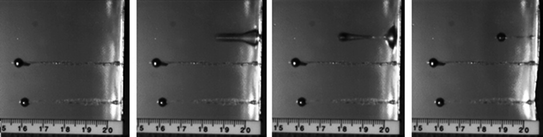
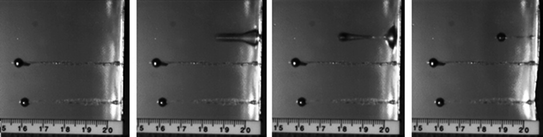
Fig. 10.2
Spherical 4.5 mm BB impacting gelatin at 65 m/s, 2 previous impacts are evident in the images. Projectile travelling from right to left
Two aspects of this impact scenario are of note: the formation and size of the temporary cavity, which is related to the amount of energy imparted to the tissue or tissue surrogate by the projectile; and the permanent cavity corresponding to localized cell necrosis (Mahoney et al. 2005). Penetrating impacts in soft tissues leads to the formation of a temporary cavity, followed by the collapse of this cavity to the wound tract or permanent cavity (Fig. 10.3). The relative size of these cavities depends on the projectile construction and velocity, and has been investigated for different projectiles (Fackler 1987) with hand guns and rifles generally treated separately due to the significant difference in projectile energy. In addition, non-deforming projectiles often yaw or tumble as they pass through tissue or tissue surrogates, generating a moderate sized temporary cavity with large penetration. In contrast, projectiles that fragment or deform significantly on impact typically result in shallower penetration but typically with a larger temporary and permanent cavity. The size of the permanent cavity is essentially related to tissue interaction directly with the projectile and is on the order of a few projectile diameters for deforming projectiles, the projectile length for non-deforming projectiles that yaw, and many projectile diameters for fragmenting projectiles. It should be emphasized that the effects are very dependent on the projectile construction and impact velocity. The reader is referred to Fackler (1987) and Fackler and Malinowski (1988) and Sellier and Kneubuehl (1994) for data on various projectiles and the experimentally measured temporary and permanent cavities.
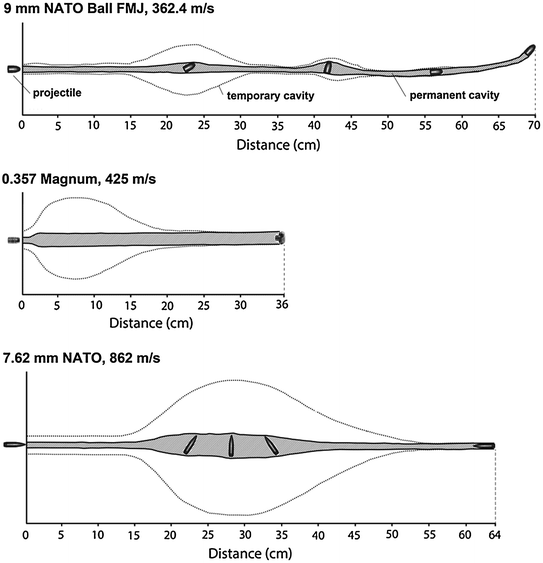
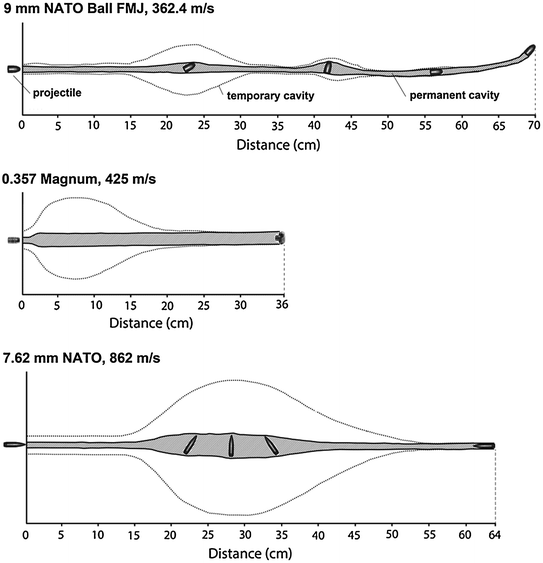
Fig. 10.3
Schematic of permanent and temporary cavities in ballistic gelatin for different projectiles (adapted from Fackler and Malinowski 1988)
Penetrating ballistic injuries in humans can result in fatalities due to collapse of the circulatory system (damage to the major vessels or heart) and/or damage to vital regions of the brain. The treatment and outcome of a penetrating ballistic injury depends greatly on timely access to appropriate care, which may be available in civilian environments but may be limited in armed conflicts, for example. Several publications addressing the treatment of ballistic injuries are available in the literature, e.g. Coupland (1993) and Molde et al. (2001). In general, soft tissue injuries are always assumed to be contaminated and are treated through debridement or opening of the wound for removal of contaminants (e.g. bullets, fragments, clothing and detached tissues). Dead or nonviable tissue is then removed or excised, the wound is irrigated and closure is delayed to ensure all contamination and nonviable tissues are removed to mitigate infection and the possibility of compartment syndrome (Mahoney et al. 2005). Impact on hard tissues often results in local bone fracture and many small bone fragments, where unattached fragments should be removed from the wound. Deformation or yaw (tumbling) of the projectile can lead to internal damage and a large exit wound relative to the entrance wound. As in other areas of injury biomechanics, ballistic injury is often discussed in terms of the body region(s) affected: head, neck, thorax, spine, abdomen and pelvis, and the limbs. Penetrating head injuries are classified as tangential (a glancing impact that may fracture the skull and cause laceration or contusion of the brain tissue), penetrating (projectile penetrates the skull resulting in contusion, laceration and hematoma) and perforating (project penetrates and exits the head), where the latter generally results in the largest amount of damage. Penetrating neck injuries can be serious, particularly if major vessels or the airway are affected, and most often require immediate treatment. Penetrating wounds to the thorax are often serious due to the potential for pneumothorax, hemothorax, pulmonary laceration and contusion, cardiac injury, and large vessel injury. The organs of the abdomen (e.g. kidney, spleen, liver) may experience large amounts of disruption and damage from penetrating gunshot wounds and require surgical intervention. Although limb wounds are one of the most common gunshot wounds, they are often not life threatening unless a major vessel (e.g. femoral artery) or a large bone (e.g. femur) is significantly damaged; however, there is a possibility for amputation in this type of injury depending on the extent of the wound and damage to vessels and/or nerves. Given the severity of penetrating injuries, anticipated exposure by police or military personnel is mitigated through the use of Personal Protective Equipment.
10.1.2 Personal Protective Equipment (Ballistic Protection and BABT)
Personal Protective Equipment (PPE) refers to worn apparel or carried items designed to protect the user from injury or trauma. Protection for the human body has historically evolved to meet changing threats to the body, including blunt impact protection using thick layers of animal hides followed by metallic armour to protect against piercing weapons. However, existing protection was rendered ineffective following the development of firearms and significant efforts were undertaken to address this new threat using various materials including metals, silk and nylon. PPE intended for protection from ballistic impacts must often protect against other forms of injury such as blunt impact from objects or falls, and other penetration threats such as knives. For the purposes of this section, the discussion is focused on soft or flexible body armour (ballistic vests) and hard body armour that may be worn separately or in conjunction with soft body armour to protect the vital organs in the thorax. However, ballistic head protection is often worn, as well as additional armour to protect the lateral aspects of the thorax, neck, groin, extremities and eyes/face.
The primary trade-offs in PPE performance include weight (mobility, ergonomics and coverage) and protection level. For armour, weight is normally expressed in terms of areal density (e.g. kg/m2) or weight per unit area so that different protections can be compared on a coverage basis. Thoracic protection is typically evaluated based on the ability to mitigate projectile perforation, and the amount of dynamic deformation that occurs during an impact, which can lead to blunt trauma. This is known as Behind Armour Blunt Trauma (BABT) (Knudsen 2010). Perforation describes a projectile that has completely passed through a target whereas projectiles that are stopped within the target are termed partial penetrations.
Soft or flexible body armour (vest) typically consists of multiple layers of ballistic fabric that allow for some flexibility and is often worn as a vest covering the thorax with areal densities up to 8 kg/m2 depending on the material used and level of protection. Modern flexible armour originated with the development of aramid fibres in the mid-1970s (e.g. Kevlar®) which was woven into fabric layers (see background of (Fig. 10.4) and used in the form of multiple stacked layers to provide ballistic protection. Various forms of aramid fibres and weaves, including uni-directional fibres have been used in flexible armour. More recently, polyethylene-based fibres (e.g. Dyneema®) have been used to provide lower areal density solutions.
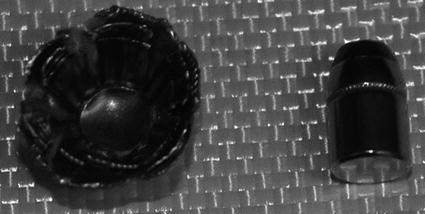
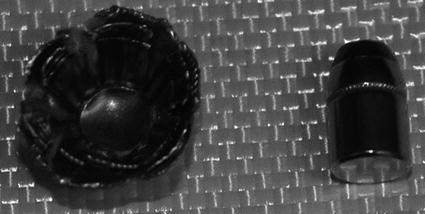
Fig. 10.4
9 mm projectile deformed after impact (left) and original (right), impact on flexible body armour
Flexible protection is intended for relatively low energy projectiles including handgun or pistol projectiles, and works by decelerating a projectile over a very small distance. An example of a 9 mm projectile pre and post impact is shown in Fig. 10.4. The deformation of projectiles is often very large with material failure occurring at high deformation rates, making modelling of these impacts challenging.
Hard or plate armour is designed to stop high energy threats that may include armour piercing cores. One example is the M2 AP projectile that comprises a gilding copper jacket, hardened steel core and lead tip filler, which is widely used in armour testing and research studies. When impacting at high velocity, it is challenging to stop this type of projectile with traditional flexible armour while maintaining acceptable weight and mobility. Hard armour is commonly constructed (Fig. 10.5) with a high hardness material on the impact or strike face of the armour to disrupt the projectile, and backed ductile or deformable material to decelerate and stop the impact debris.
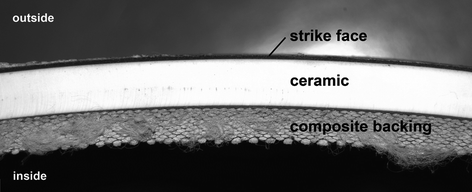
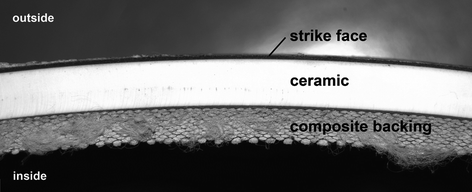
Fig. 10.5
Conventional hybrid hard armour cross-section
The impact of a projectile on ceramic with a thin backing has been described by several authors (Wilkins 1978; den Reijer 1991) and begins with the initial contact between the projectile and ceramic blunting the projectile and initiating a shock wave in the ceramic (Fig. 10.6). This is known as the dwell stage and occurs over a time of 6–8 μs. As the impact progresses, damage is initiated in the ceramic at the impact location and fractures propagate outwards creating a fracture conoid (Fig. 10.7). Movement or flow of the comminuted ceramic allows for ingress of the projectile while continuing to erode and deform the projectile (Fig. 10.8). As the impact proceeds, the backing material begins to deform, decelerating the projectile and impact debris. Depending on the armour construction, projectile construction, and impact velocity the projectile and debris may be stopped in the armour or may perforate the armour.
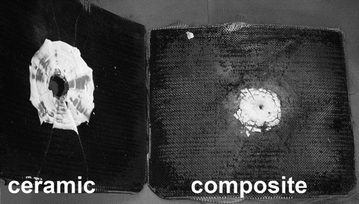
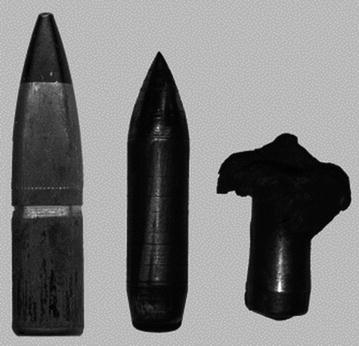
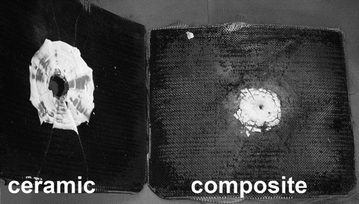
Fig. 10.7
Conventional hybrid armour opened to show the fracture conoid on the back of the ceramic and composite backing which has stopped the impact debris
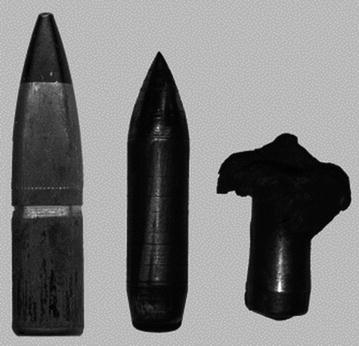
Fig. 10.8
M2 AP projectile (left), intact steel core (centre), and steel core following impact with hard armour (right)
The impact or strike face is often constructed from ceramic materials due to their high hardness and strength, with a covering or spall cover to mitigate the expulsion of impact debris and improve the ceramic performance. The purpose of this hard strike face is to break up or disrupt the projectile and the ceramic is often fractured or damaged during the impact event. To a large extent, the amount of damage in the ceramic determines the viability of the armour to withstand multiple projectile impacts, also known as multi-hit capability. Common ballistic ceramics include Aluminum Oxide, Boron Carbide and Silicon Carbide. The backing material typically consists of ballistic fabric layers that are laminated or bonded together using a thermoset (e.g. PVB phenolic) or thermoplastic (e.g. polyethylene-based) matrix to enhance penetration resistance.
At typical projectile impact velocities the pressures generated at the impact site are immense and the equation of state (relationship between pressure, volume and energy) must be considered to describe the material response (Meyers 1994). Interesting phenomena may occur at the impact site such as spallation, where a compressive stress wave encounters an interface of lower impedance and is reflected as a tensile wave causing material damage, and bulking where the fractured or comminuted ceramic volume is larger than the intact material volume.
10.1.3 Armour Performance and Testing
The performance of body armour is assessed on the ability to resist perforation and the amount of dynamic deformation during the impact, also known as Back Face Signature (BFS). An example of a widely used test standard is the National Institute of Justice (NIJ) Standard-0101.06 (NIJ 2008) Ballistic Resistance of Body Armour, which provides testing and performance standards for body armour. In this standard, body armour is classified according to types depending on the anticipated threat or projectile type and velocity. Note that armour is not defined as “bulletproof” but as ballistic resistant based on the specified projectile type and velocity. Armour may be tested in the as-manufactured or “as-new” state (Table 10.1), and in a conditioned state (Table 10.1) where it has been exposed to environmental conditioning (for example 65 °C temperature, exposure to 80 % relative humidity, and tumbling exposure of 72,000 rotations) to simulate the effect of time and exposure for soft or flexible armour. Similarly, hard armour conditioning requires exposure to temperature and humidity as well as thermal cycling (−15–90 °C) and drop testing, which may affect bonding within the plate or the integrity of the ceramic component.
NIJ level | Projectile | Mass (g) | Impact velocity (m/s) |
---|---|---|---|
For as-new armour: | |||
Type IIA | 9 mm FMJ RN | 8.0 | 373 ± 9.1 |
0.40 S &W FMJ | 11.7 | 352 ± 91 | |
Type II | 9 mm FMJ RN | 8.0 | 398 ± 9.1 |
0.357 Magnum JSP | 10.2 | 436 ± 9.1 | |
Type IIIA | 0.357 SIG FMJ FN | 8.1 | 448 ± 9.1 |
0.44 Magnum SJHP | 15.6 | 436 ± 9.1 | |
Type III (rifles), hard armoura | – | – | – |
Type III (rifles), flexible armour | 7.62 mm FMJ (M80) | 9.6 | 847 ± 9.1 |
Type IV (armour piercing rifle), hard armoura | – | – | – |
Type IV (armour piercing rifle), flexible armour | 0.30 calibre armour piercing (M2 AP) | 10.8 | 878 ± 9.1 |
Special typeb | Defined by purchaser in the case of special requirements based on the threat or protection performance. | ||
For conditioned armour: | |||
Type IIA | 9 mm FMJ RN | 8.0 | 355 ± 9.1 |
0.40 S & W FMJ | 11.7 | 325 ± 9.1 | |
Type II | 9 mm FMJ RN | 8.0 | 379 ± 9.1 |
0.357 Magnum JSP | 10.2 | 408 ± 9.1 | |
Type IIIA | 0.357 SIG FMJ FN | 8.1 | 430 ± 9.1 |
0.44 Magnum SJHP | 15.6 | 408 ± 9.1 | |
Type III (rifles), hard armoura | 7.62 mm FMJ (M80) | 9.6 | 847 ± 9.1 |
Type III (rifles), flexible armour | 7.62 mm FMJ (M80) | 9.6 | 847 ± 9.1 |
Type IV (armour piercing rifle), hard armoura | 0.30 calibre armour piercing (M2 AP) | 10.8 | 878 ± 9.1 |
Type IV (armour piercing rifle), flexible armour | 0.30 calibre armour piercing (M2 AP) | 10.8 | 878 ± 9.1 |
Special typeb | Defined by purchaser in the case of special requirements based on the threat or protection performance. |
The obvious goal of ballistic protection is to prevent armour perforation, which can then lead to penetrating injury for the wearer. However, even when the projectile is stopped in the armour (partial penetration) the dynamic deformation of the armour during the impact must be minimized to protect the vital organs in the thorax. Significant dynamic deformation can lead to blunt thoracic trauma (Behind Armour Blunt Trauma, BABT). The extent of BABT will depend on the rate of impact and extent of deformation.
The performance of body armour is typically rated using the National Institute of Justice standard which places limits on the penetration resistance of the armour, measured by V50, the velocity at which 50 % of the projectiles are expected to penetrate the armour. Other measures, such as V05 corresponding to lower probability of perforation may also be specified in test standards. Ballistic testing may be undertaken with the armour mounted on a special type of clay backing (Fig. 10.9) which is used to record the dynamic deformation of the armour. The clay is specified as Roma Plastilina No.1 oil-based modelling clay and must be at a specific temperature and calibrated with a steel ball drop test to be used for ballistic testing. In the NIJ standard, the measured dynamic deformation in the clay is limited to 44 mm while other specifications may use different values, depending on the protection level. For example, the UK police test standard uses an upper prediction limit of 25 mm for the Back Face Signature (BFS) (Croft and Longhurst 2007). It should be noted that the methods for calculating V50 and BFS may differ for different test standards, and may include statistical methods using multiple impacts to determine the likelihood of perforation or maximum BFS.
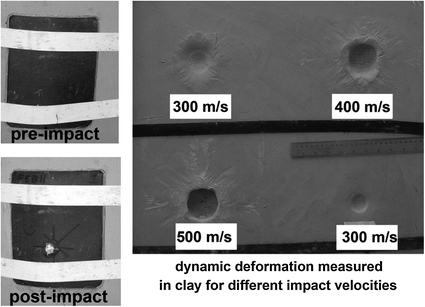
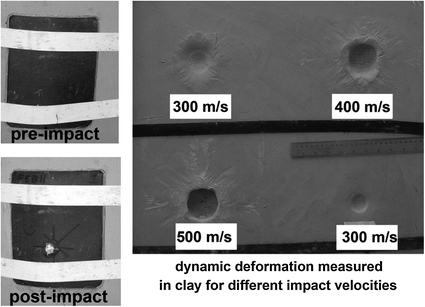
Fig. 10.9
Impact testing of armour on clay (left) and resulting deformation in clay (right)
10.2 Blast Injury and Protection
Exposure to blast in the civilian environment is associated with accidental exposure (e.g. dust, fertilizer plant, natural gas explosions) and intentional exposures (e.g. Oklahoma city bombing 1995, Boston Marathon bombing 2013). Although many different types of explosives exist the output of an explosion is often characterized in terms of Trinitrotoluene (TNT) equivalent with values for conventional high explosives ranging from a few grams to several kilotons. For example, the 1917 Halifax harbour explosion of approximately 2.9 kilotons TNT equivalent (Glasner 2007) was the largest man-made explosion prior to the development of nuclear weapons. In comparison, the Hiroshima nuclear bomb yield was approximately 13 kilotons. In conflicts, blast exposure from conventional weapons and Improvised Explosive Devices (IEDs) has become more common with many IEDs in the range of 12–23 kg (Flynn 2009) and some exceeding 45 kg. However, typical human exposures to blast are on the order of tens of kilograms of TNT equivalent (Haladuick et al. 2012) and the severity of exposure depends on the distance between the explosive and target, level of protection worn, and surrounding environment.
10.2.1 Explosives and Detonation
An explosion is a rapid release of energy, which is typically adiabatic over the short duration of the explosion. Explosions can be classified as physical (e.g. pressure vessel failure), nuclear and chemical. A chemical explosion occurs by fast oxidation of an explosive material producing heat and gases which expand rapidly and generate shock waves in the expansion medium. Similar to ballistics, the development of chemical explosives began with the invention of gunpowder or black powder (potassium nitrate, carbon and sulphur). This was followed by the creation of various explosives based on nitric acid, the invention of nitroglycerine, the invention of dynamite by Alfred Nobel, and finally the development of TNT, the latter of which could be manufactured relatively safely and cheaply and became the standard explosive during WWI. More recent explosives include RDX (cyclonite or cyclotrimethylenetrinitramine), which is a common component used in many explosives such as C-4, which is often described as a plastic explosive. C-4 (91 % RDX) has a TNT equivalent of approximately 1.34 (Department of the Army 1967), based on pressure. It should be noted that the equivalence values may vary between literature sources and depend on the specific equivalence method (e.g. pressure or impulse).
Rapid reactions that convert explosive material into gases can be classified as deflagration or detonation. Deflagration or burning is a slow chemical conversion of explosive material into products and commonly refers to a reaction between fuel and atmospheric air but can produce an explosion if contained. An example is a dust explosion where a material such as coal, sawdust, grain and flour can produce dust clouds from manufacturing or handling processes. If a combustible dust material in high concentration, with particles generally less than 75 μm in size (Hetherington and Smith 1994), is suspended in the air with sufficient oxygen an ignition source can result in deflagration of the suspended material. In a case where the material is contained, such as in a building, the resulting increase in pressure can lead to an explosion and destruction of the structure. This same principle is often used in thermobaric weapons, which can generate relatively long duration overpressures using a fuel (e.g. powdered metal) dispersed over a large area which is ignited and relies on oxygen in the atmosphere for combustion.
In contrast, detonation requires the propagation of a shock wave through the explosive to provide the necessary activation energy for the chemical reaction (Fig. 10.10), and the explosive typically incorporates the necessary amount of oxygen required for the chemical reaction. This shock wave is typically provided by a detonator, which may utilize the vaporization of a wire to initiate the reaction. Explosives that require a significant amount of energy to detonate are known as high explosives (HE).
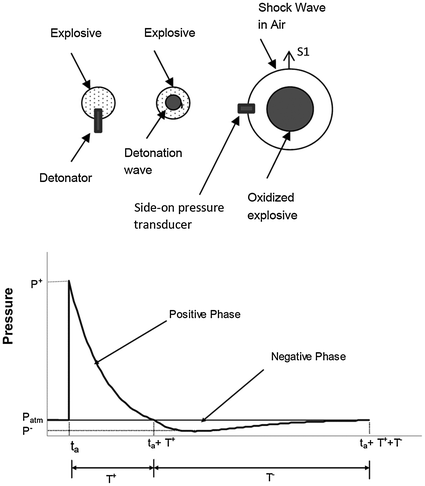
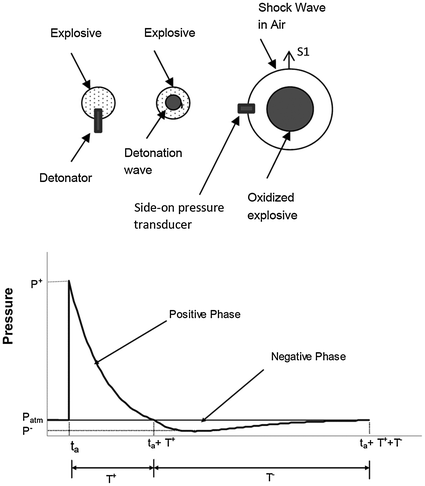
Fig. 10.10
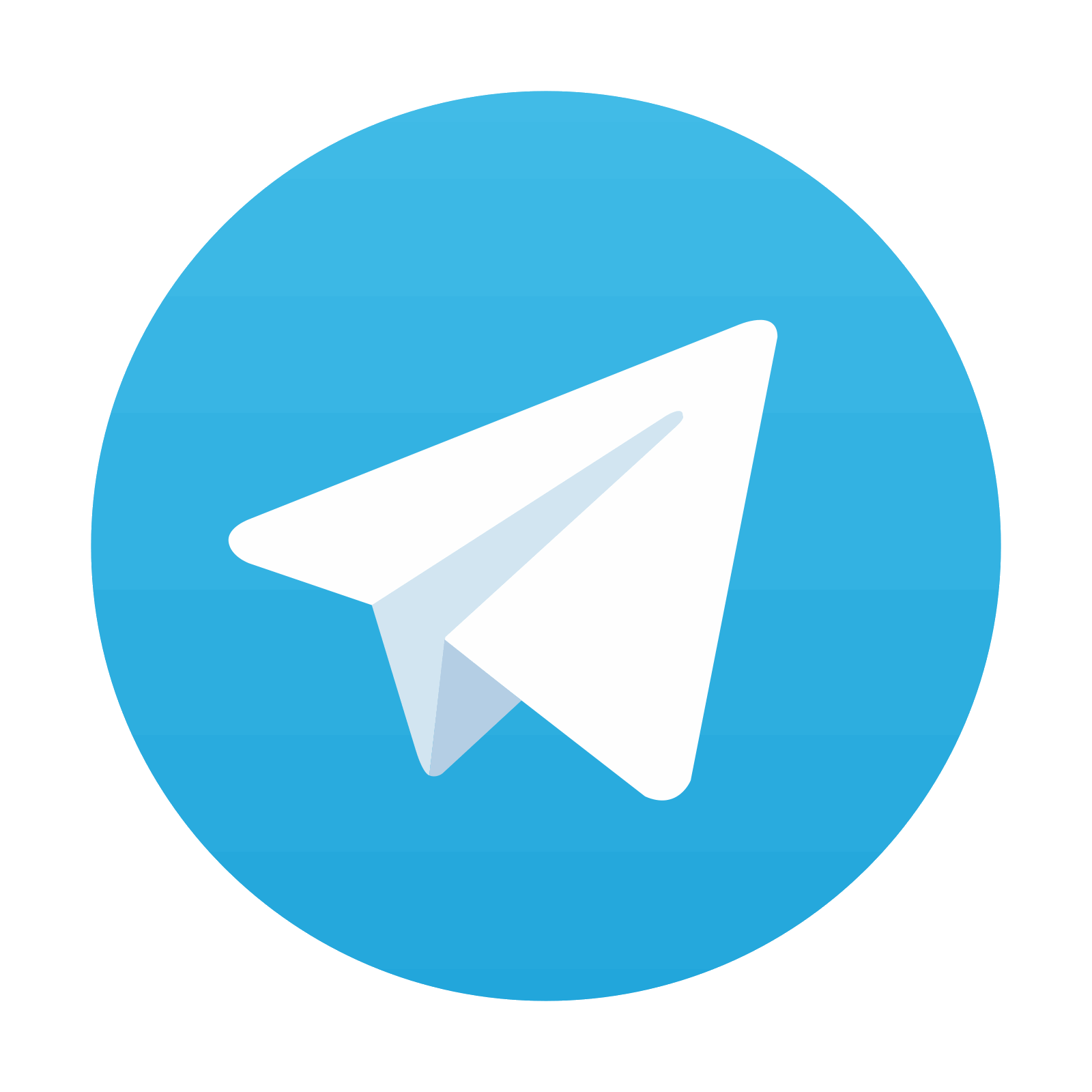
Detonation of an explosive (top) and resulting free-field pressure wave (bottom)
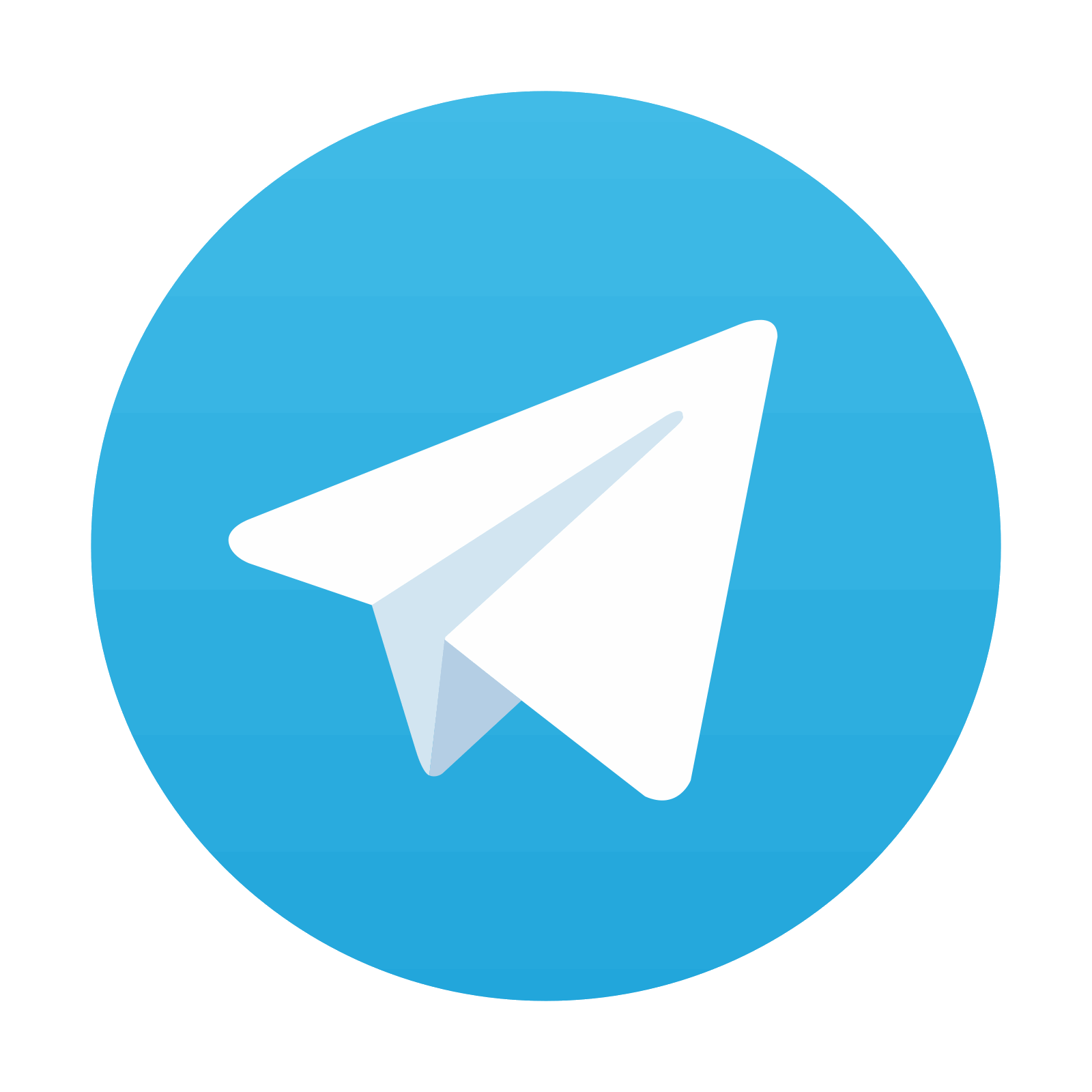
Stay updated, free articles. Join our Telegram channel
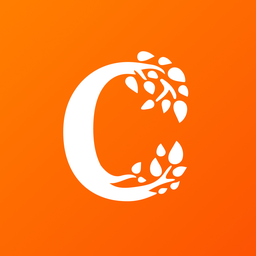
Full access? Get Clinical Tree
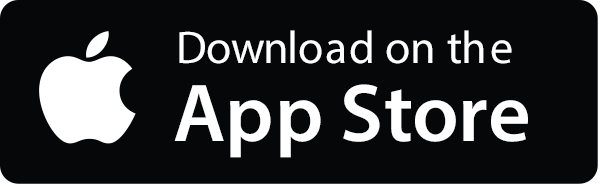
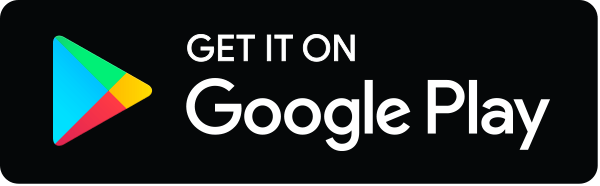
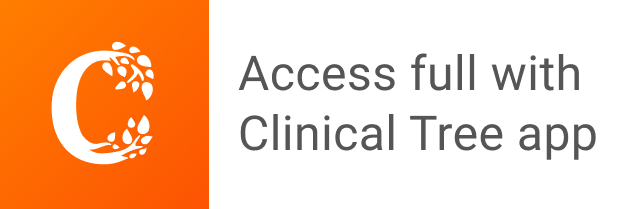