24 Anesthetic-induced Neurotoxicity
Andreas W. Loepke and Mary Ellen McCann
II. Effects of exposure to anesthetics, sedatives, and analgesics on the developing animal brain
A. Deleterious effects of anesthetic exposure
B. Effects of opioid exposure on brain development
C. Anesthesia-induced neuroprotection
III. Effects of untreated pain and stress on the newborn animal brain
A. Deleterious effects of noxious stimulation
IV. Possible mechanisms of anesthetic-induced neuronal cell death
A. Abnormal neuronal inhibition
C. Interference with trophic factors
V. Potential mitigating strategies
D. Alternative anesthetics/sedatives
VI. Human applicability of animal data
A. Experimental versus clinical conditions
B. Exposure time relative to biologic events
C. Equivalency of anesthetic drug doses
D. Comparative brain developmental states
E. Assessment of neurocognitive outcomes
VII. Outcome in children exposed to surgery with anesthesia
A. Cohort studies of prenatal exposure
B. Cohort studies of neonatal and early childhood exposure
C. Epidemiologic studies specifically examining anesthetic exposure
KEY POINTS
1. Prolonged exposure to most general anesthetics and sedatives administered to young mammals, including NMDA inhibitors (ketamine, nitrous oxide) and GABAA agonists (volatile anesthetics, barbiturates, benzodiazepines, propofol), has produced neurotoxic effects.
2. Detrimental effects have been observed on neuronal survival, neuro- and gliogenesis, dendrite formation, and long-term neurocognitive function.
3. Deleterious consequences are dose dependent and most often occur following prolonged or repeated exposures to general anesthetics.
4. Prolonged opioid exposure early in life can mimic many of the deleterious effects of general anesthetics; however, the comparative toxic potency remains unclear.
5. These abnormal findings peak during very early stages of brain development. The equivalent developmental state of the human brain remains controversial, complicating easy human translation of animal studies.
6. Several human epidemiologic studies have shown a link between anesthetics before the age of 4 years, and developmental and behavioral abnormalities later in childhood.
7. At this point in time, there is not enough evidence for general anesthetic neurotoxicity in humans to recommend any changes in clinical practice.
I. Introduction. General anesthetics have been used for more than 160 years to facilitate surgery and to mitigate distress during painful procedures. Thus, millions of children worldwide are exposed to these agents every year. However, animal studies have uncovered potentially deleterious effects of prolonged anesthetic exposure on the developing brain [1,2]. While translation of these animal studies to pediatric anesthesia practice remains very difficult, the findings have led to serious concerns regarding the safe use of anesthetics in small children.
II. Effects of exposure to anesthetics, sedatives, and analgesics on the developing animal brain
1
A. Deleterious effects of anesthetic exposure. Thus far, over 250 studies in immature animal models, including chicks, mice, rats, guinea pigs, pigs, and nonhuman primates, have shown exposure to anesthetic drugs and sedatives, such as desflurane, enflurane, halothane, isoflurane, sevoflurane, nitrous oxide, chloral hydrate, clonazepam, diazepam, ketamine, midazolam, pentobarbital, phenobarbital, and propofol may be deleterious (reviewed in [3]).
2
1. Apoptotic cell death (and long-term viability). Apoptosis, or programmed cell death, which is a normal part of mammalian development, is increased following exposure to anesthesia in many immature animals.
a. Normal neuroapoptosis eliminates up to 70% of all brain cells during development.
b. The period of maximum susceptibility to anesthetic-induced neuroapoptosis is species specific, but generally corresponds to early stages of brain development.
c. The window of vulnerability can be quite narrow. For example, rat pups younger than 1 day of age or older than 10 days are seemingly unaffected by anesthetic-induced neuroapoptosis in several forebrain structures [4].
3
d. Anesthetic-induced neuroapoptosis is dose- and exposure time-dependent.
e. The long-term effects of anesthetic-induced neurotoxic injury on subsequent neuronal density is controversial. One rat study showed a permanent decrease in neuronal density in adult rats, but another study showed no diminution, suggesting species- or drug-specific variability.
f. Long-term neurocognitive impairment has been observed in several animal studies, while other studies did not detect neurologic abnormalities. Furthermore, most of the studies showing impairment have demonstrated neurocognitive deficits in discrete domains of testing, especially hippocampal learning, rather than global deficits.
2
2. Neurogenesis and gliogenesis
a. Abnormalities in neurogenesis—both reductions in neuronal stem cells and decreases in neuronal proliferation— have been observed in immature rats following prolonged exposure to isoflurane.
b. Exposure to 24 hours of 3% isoflurane has been shown to impair growth and to delay maturation of astrocytes.
2
3. Dendritic architectural alterations
a. Ketamine, midazolam, propofol, desflurane, isoflurane, and sevoflurane can alter dendritic branching and synaptic density.
b. Exposure to anesthesia during the first 2 weeks of life decreases synaptic and dendritic spine density whereas exposure after 2 weeks increases the number of dendritic spines in rodents [5].
c. The functional significance of these findings and their permanence are unknown, with some studies showing no lasting effects later in life.
4
B. Effects of opioid exposure on brain development. One study comparing an isoflurane-based anesthetic with a high-dose fentanyl anesthetic in newborn piglets found a dramatically reduced rate of neuroapoptotic cell death using the opioid versus isoflurane [6]. However, while not as widely studied as anesthetics, prolonged opioid exposure in immature animals can also lead to neurologic abnormalities.
1. Opioid-induced apoptotic cell death. Exposure to opioid receptor agonists in immature neurons can lead to several abnormalities (see [3] for details), including mitochondrial dysfunction, diminished neuronal viability, increased apoptosis, and DNA fragmentation. Moreover, apoptotic cell death has also been observed in neurons and microglia, but not astrocytes in human fetal brain cell cultures.
2. Long-term neurologic functional impairments. Several studies have observed long-term learning impairment in adult animals following prolonged morphine exposure early in life. Moreover, altered pain responses in adulthood were linked to exposure to the opioid agonists morphine, fentanyl, or methadone, early in life.
3. Abnormal alterations in neurotrophic factors. Similar to anesthetics, opioid receptor agonists, including buprenorphine and methadone, have also been found to decrease nerve growth factors in the immature brain.
4. Alterations in opioid receptor density. Diminished μ-receptor densities have been demonstrated immediately following prolonged morphine exposures in immature rats, and have been found to extend into adulthood.
C. Anesthesia-induced neuroprotection
1. Many general anesthetics (propofol, ketamine, isoflurane, sevoflurane, and xenon) have been shown to have protective effects in neuronal preservation in the setting of hypoxia and brain ischemia in adult animals.
2. Several studies using immature brain ischemia models have shown an improvement in neuronal survival by anesthetics [7,8].
CLINICAL PEARL
It is important to remember that untreated pain also causes neurologic abnormalities in animals and humans, demanding adequate anesthesia and analgesia in young children undergoing painful procedures.
III. Effects of untreated pain and stress on the newborn animal brain. Extensive evidence from laboratory and clinical studies has demonstrated that unopposed pain and stress can initiate deleterious effects in the developing brain that may be long lasting.
A. Deleterious effects of noxious stimulation. Painful stimulation and separation stress have been found to not only cause short-term alterations in the immature brain, but also to carry long-term effects into adulthood. Moreover, administration of anesthetics/analgesics may be able to diminish some of these deleterious effects caused by pain.
1. Apoptotic cell death. Immediately following repeated painful injections, extensive neuronal cell death was observed in neonatal rats [9]. Preemptive analgesia with small doses of ketamine ameliorated the deleterious effects of unopposed pain [9].
2. Long-term neurologic consequences
a. Repetitive painful skin lacerations can lead to long-term, local sensory hyperinnervation.
b. Inflammatory pain early in life resulted in hyperalgesia and lasting changes in nociceptive circuitry of the adult dorsal horn. Moreover, rat pups receiving repeated painful injections into the paw subsequently developed a generalized thermal hypoalgesia.
c. Altered behavior and cognitive function, decreased pain thresholds, increased vulnerability to stress and anxiety disorders or chronic pain syndromes have all been observed in adult animals exposed to painful stimulations early in life.
d. In addition to pain, adverse emotional experiences early in life can also induce long-lasting abnormalities, such as imbalances of the inhibitory nervous system, impairment of normal development of the nociceptive system, long-term behavioral changes, and persistent learning impairment.
IV. Possible mechanisms of anesthetic-induced neuronal cell death. Several hypotheses have been proposed to explain the neurotoxic effects of anesthetics, especially neuroapoptosis. Apoptotic cell death is vital to brain development to rid the organism of abnormal or superfluous cells and to prevent tumor formation. Numerous pathways, both promoting and preventing apoptosis, need to be balanced to promote cellular survival; anesthetics may interfere with several of these pathways, thereby altering this equilibrium and ultimately leading to cell death.
A. Abnormal neuronal inhibition. The most commonly advanced hypothesis for the mechanism of anesthetic neurotoxicity is that of an anesthesia-induced inhibition at the NMDA receptor and/or stimulation at the GABAA receptor leading to abnormal inhibition in immature neurons during a vulnerable developmental period, thereby triggering the cell’s inherent apoptotic cell death machinery [10]. Incidentally, both GABA and NMDA receptor-mediated activities are essential during brain development. However, while NMDA-antagonist xenon and hypothermia increase the depth of anesthesia and thereby neuronal inhibition, they do not increase neuronal cell death, but may actually protect from the neurotoxic effects of other anesthetics [11,12].
B. Excitotoxicity
1. Another theory posits that an upregulation of NMDA receptor expression during a prolonged exposure to an NMDA antagonist, such as ketamine, exposes neurons to excitotoxic injury by endogenous glutamate immediately following the anesthetic’s withdrawal [13].
2. In the immature brain, GABA is an excitatory rather than inhibitory neurotransmitter. Accordingly, GABA-ergic agents may cause excitation and even seizures due to the elevated intracellular chloride concentration resulting from the immature chloride transporter NKCC1, which produces chloride efflux leading to cell depolarization. GABA switches to its inhibitory mode once expression of the mature chloride transporter KCC2 intercedes.
C. Interference with trophic factors. The trophic factor BDNF is integral to neuronal survival, growth, and differentiation. Isoflurane-induced apoptosis in newborn mice has been found to be triggered by interference with BDNF formation [14].
D. Re-entry into cell cycle. Some experimental models of neurodegeneration have implicated the re-entry of postmitotic neurons into the cell cycle in contributing to cell death. Accordingly, ketamine exposure has been found to induce aberrant cell cycle re-entry, leading to apoptotic cell death in the developing rat brain.
E. Mitochondrial function. Anesthetics have recently been found to impair mitochondrial morphogenesis, to decrease mitochondrial density, and to lead to long-lasting disturbances in inhibitory synaptic transmission following a combined exposure to midazolam, nitrous oxide, and isoflurane in newborn rats.
F. Cytoskeletal integrity. Cytoskeletal destabilization as well as neuronal and astroglial depolymerization of actin, a major component of the cytoskeleton of all eukaryotic cells that participates in important cellular processes, such as cell signaling, cellular division, and motility as well as dendrite formation, have been detected in immature rodents following isoflurane exposure and may play a role in the observed apoptotic brain cell death.
V. Potential mitigating strategies. Since all currently routinely used anesthetics have demonstrated neurotoxic properties, extensive research efforts have been spent on finding alternative agents or mitigating treatments.
A. Preconditioning. Anesthetics, including isoflurane, can diminish cellular injury when administered briefly prior to an ischemic insult. This concept, called preconditioning, was also demonstrated by a short preconditioning exposure to isoflurane in rat’s primary cortical neurons 4 hours prior to a prolonged exposure, which protected from the deleterious effects of the subsequent prolonged anesthetic exposure.
B. Protective drug therapy. Several drugs and endogenous hormones have been tested in conjunction with anesthetics to examine their protective properties for anesthesia-induced neurotoxicity.
1. Caffeine. In order to provide respiratory and neurologic stimulation during anesthetic exposure, caffeine has been studied as a protective agent during isoflurane exposure in newborn mice. However, preliminary results suggest that caffeine augmented, rather than ameliorated, the isoflurane-induced neuroapoptosis and also promoted neuronal cell death when administered by itself, questioning its role as a protective agent.
2. L-carnitine. L-carnitine was able to attenuate neuronal apoptosis caused by a 6-hour exposure to isoflurane and nitrous oxide in 7-day-old rat pups.
3. b-estradiol. The deleterious effects of a prolonged exposure to midazolam, isoflurane, and nitrous oxide on neuronal survival were successfully treated with β-estradiol supplementation. Similarly, phenobarbital-induced neuroapoptosis was significantly reduced by coadministration of β-estradiol in young rats.
4. Melatonin. Administration of the natural hormone melatonin was able to protect from the deleterious effects of the combination exposure to midazolam, isoflurane, and nitrous oxide in newborn rats.
5. Jasplakinolide or TAT-Pep5. Inhibition of the RhoA receptor or prevention of cytoskeletal depolymerization with either jasplakinolide or TAT-Pep5 has been found to attenuate isoflurane- or propofol-mediated neuroapoptosis.
6. Lithium. A protective strategy successfully tested in mouse pups exposed to ketamine or propofol was lithium, which abolished the anesthetic-induced neuroapoptosis in cortex and caudate/putamen. However, lithium has been labeled harmful to the human fetus and may cause neurocognitive impairment in young children.
7. Pilocarpine. Preliminary results in neonatal mice seem to suggest that pilocarpine may reduce neuroapoptosis induced by isoflurane and midazolam, while augmenting neurotoxicity caused by the NMDA-antagonist phencyclidine. However, pilocarpine’s safety in young children is questionable due to its proconvulsant activity observed in animal studies.
8. tPA, plasmin, p75NTR inhibition. In the context of increasing the trophic factor BDNF, the coadministration of tissue-plasminogen activator, plasmin, or the pharmacologic inhibition of the neurotrophic receptor p75NTR during isoflurane exposure has been successfully studied to prevent isoflurane’s neurotoxic effects [16].
9. Xestospongin C. In an in vitro study, the IP3 receptor-antagonist xestospongin C significantly ameliorated isoflurane cytotoxicity in primary cortical neurons.
10. Bumetanide. The diuretic bumetanide significantly decreased seizures in immature rats and caused a significant decrease in expression of activated caspase-3, a marker for apoptosis.
C. Hypothermia. Whole-body hypothermia of less than 30°C may protect from the neuroapoptotic ramifications of isoflurane or ketamine in neonatal mice. There are, however, significant difficulties in administering hypothermia to human infants including increased blood loss, prolonged postanesthetic recovery, and increased risk of surgical site infections.
D. Alternative anesthetics/sedatives
1. Xenon. The NMDA-antagonist xenon has been studied regarding its neurotoxic potential and has been found to only hold limited toxic potency [12]. Moreover, when administered in conjunction with isoflurane, xenon was able to mitigate some of the volatile anesthetic’s toxic effects [12]. However, xenon has limited anesthetic potency compared with volatile anesthetics and due to its scarcity, remains very expensive.
2. Dexmedetomidine. The α2-agonist dexmedetomidine has sedative properties and has been investigated regarding its toxic and protective properties in the developing brain. The encouraging finding from thus far limited in vitro and in vivo studies has been that repetitive injections of dexmedetomidine did not cause any neuroapoptosis and inhibited isoflurane-induced neuroapoptosis [15]. Moreover, dexmedetomidine also prevented memory impairment caused by isoflurane. However, because of its limited anesthetic potency, dexmedetomidine cannot be used as a sole anesthetic agent.
3. Ketamine. When given in large doses, or repeatedly over a prolonged period of time, ketamine is associated with marked apoptotic neuronal cell death. However, in lower analgesic doses, this NMDA antagonist ameliorated the immediate neuroapoptotic response to repetitive inflammatory pain and prevented subsequent pain-induced neurocognitive deficits [9]. Moreover, ketamine as well as other anesthetics have also been found to promote prosurvival cell proteins and to protect neurons exposed to ischemic stresses.
VI. Human applicability of animal data. The human applicability of the disturbing findings of anesthetic neurotoxicity in animal models remains unclear. Several differences exist between animal studies and pediatric anesthesia practice.
A. Experimental versus clinical conditions. Experimental conditions deviate from clinical practice in a variety of factors. Thus far, no animal model has been developed that completely replicates conditions during pediatric surgery and anesthesia.
1. Airway management. A majority of animal models rely on spontaneous breathing with a natural airway and not the instrumented airway, and controlled ventilation used during pediatric surgery, predisposing animals to adverse respiratory events.
2. Physiologic monitoring. Continuous physiologic monitoring, including electrocardiography, pulse oximetry, capnography, as well as temperature and blood pressure measurements are not routinely performed during animal studies, especially not in small rodent models. Several animal studies have shown abnormal blood gas analyses such as metabolic and respiratory acidosis.
3. Exposure times. Exposure times in animal studies are often protracted exposures of up to 24 hours. These studies may be more relevant to human intensive care sedation practices rather than the operating room.
B. Exposure time relative to biologic events
1. Relative life expectancies. Some suggestions exist that expressing the anesthetic exposure time as a fraction of the organism’s life span would approximate a 6-hour anesthetic exposure in mice to an anesthetic lasting more than 2 weeks in humans. However, this argument is probably an oversimplification, given the fact that the rate of cellular processes is more closely related among species.
5
2. Comparative duration of brain development. However, since human brain development occurs at a much slower pace than in any other species, similar exposure times could have different effects on potential susceptibility and ability for postexposure repair among species. For example, the brain reaches adult size at 20 days of age in rats, 3 years of age in rhesus monkeys, 7 years of age in chimpanzees, and not until 15 years of age in humans. Accordingly, given the dramatic plasticity of the developing brain, it seems conceivable that slower growth rates may leave the brain more time for repair.
3. Cell cycle duration. Even on a cellular level, significant differences exist between humans and animals; during cortical neurogenesis, cell cycle duration is approximately 17 hours in mice, 28 hours in macaque monkeys, and 36 hours in humans. It therefore seems an oversimplification to equate anesthetic exposure times 1:1 between humans and animals.
C. Equivalency of anesthetic drug doses
1. Inhalational anesthetic doses used in animal research are mostly comparable between animals and humans.
2. Injectable drug doses are typically 3- to 12-fold higher in animals to achieve similar planes of anesthesia. The dose of ketamine needed to anesthetize a rhesus monkey infant is more than 10 times of what is needed to anesthetize a human infant. Accordingly, plasma levels of drugs are much higher in the animal studies, possibly exaggerating the neurotoxic effects of intravenous anesthetics.
D. Comparative brain developmental states. The potentially vulnerable period for anesthetic-induced neuroapoptosis during human development has not been identified. Rapid brain growth occurs in humans between the third fetal trimester and the first 24 months of life. Neuroinformatic approaches for predicting human vulnerability based on animal data suggest that the human brain may be most susceptible before birth.
1. Small rodents. Peak vulnerability for several forebrain structures in rodents centers on postnatal day 7, equating to 20 to 22 weeks of gestation in human fetuses.
2. Nonhuman primates. Susceptibility to ketamine-induced neurotoxicity has been observed in macaques at 122 days of gestation and at postnatal day 5, but not in 35-day-old animals [13]. The brain maturational state in early postnatal monkeys is closer to the term and early postnatal human neonate and studies in these animals may therefore be more applicable to pediatric anesthesia than current rodent studies [17].
E. Assessment of neurocognitive outcomes
1. Assessment of neurocognitive performance in animal models largely relies on hippocampal-dependent memory tasks.
2. There, currently, is no evidence to suggest these same domains are affected in children exposed to general anesthesia.
CLINICAL PEARL
The choice of timing of surgery should depend on the child’s medical condition, the projected surgical outcomes and the immediate anesthetic concerns rather than theoretic concerns about anesthetic neurotoxicity. If parents or practitioners seek to delay truly elective surgery, unfortunately no safe duration for postponing the procedure can be rationally recommended based on the currently available data.
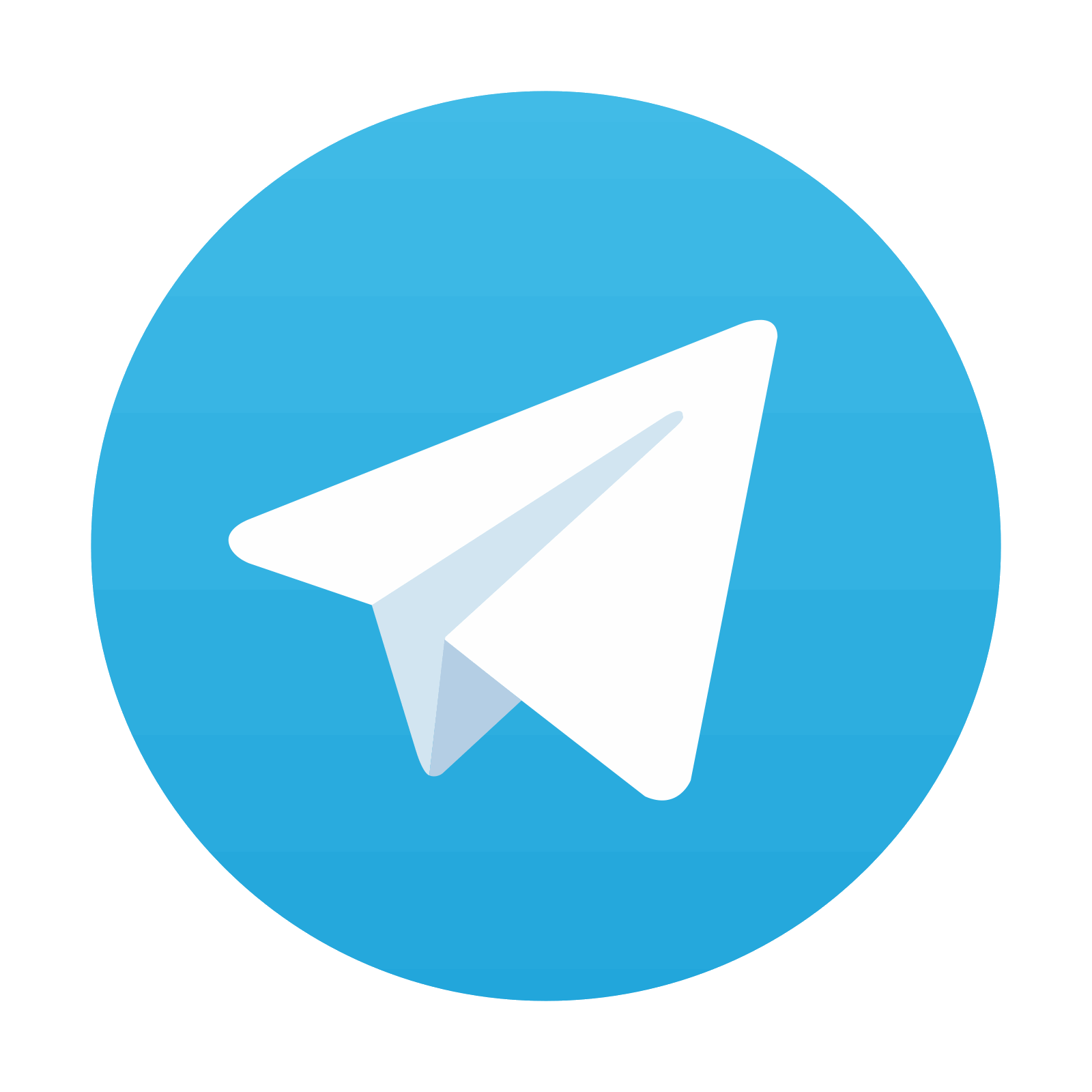
Stay updated, free articles. Join our Telegram channel
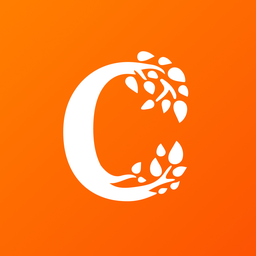
Full access? Get Clinical Tree
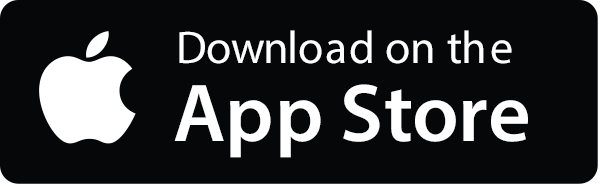
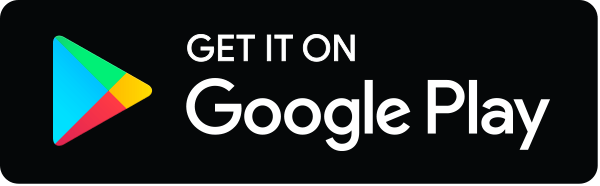