Surgical management of brain arteriovenous malformations (AVMs) is one of the most challenging in neurosurgery and, despite the relative rarity of the disease, the subject of a disproportionally large fraction of the literature on surgical cerebrovascular disease. Perioperative and anesthetic management is optimal when the anesthesiologist has familiarity with the strategic goals of therapy and familiarity with AVM pathophysiology. This chapter will summarize these topics and discuss specific neuroanesthetic issues regarding care of these patients. While the fundamentals of providing perioperative care are similar to other neurovascular conditions, there are some important considerations unique to AVMs.
Clinical behavior
Brain AVMs are a relatively infrequent, but important source of neurological morbidity in young adults. The basic morphology is of a vascular mass, called the nidus, that directly shunts blood between the arterial and venous circulations without a true capillary bed. Hemodynamic alterations include variable degrees of high flow through the feeding arteries, nidus and draining veins, as well as venous hypertension. The nidus is a complex tangle of abnormal, dilated channels, not clearly artery or vein, with intervening gliosis.
AVMs may exert a deleterious effect on brain function by several mechanisms, including mass effects (e.g., hematoma, edema or gradually expanding abnormal vascular structures such as venous aneurysms), metabolic depression (diaschisis) and seizure activity. The most common presentation and source of morbidity, however, is spontaneous intracranial hemorrhage (ICH) from rupture, occurring in about one-half of all patients.
The risk of spontaneous ICH without treatment is commonly estimated to be approximately 2–4% per year for all patients, but the rate varies widely depending on what ICH risk factors are present. The best studied risk factors are a presentation with ICH, deep location, and venous drainage pattern. , Factors such as small size of lesion and advanced age are less robust; others, such as presence of aneurysms, are harder to accurately define. , High intranidal pressure, as measured with direct puncture of feeding arteries or during superselective angiography, is associated with hemorrhage presentation.
Clinical presentation with ICH appears to be the strongest risk factor for future hemorrhage. There are a number of reports concerning other risk factors, , but few that have prospectively assessed future hemorrhage risk. , , The risk of spontaneous ICH has been estimated in retrospective and prospective observational studies to range from 2% to 4% per year. However, depending on a number of risk factors, the range of yearly bleeding risk varies widely, estimated to range from under 1% to over 30% per year.
Approximately 10% of patients with AVMs also harbor intracranial aneurysms. Note that the converse is not true; the detection rate of AVMs in aneurysm patients is closer to the detection rate of AVMs in the general population. Intracerebral hemorrhage from aneurysms is usually associated with subarachnoid hemorrhage, whereas AVMs more commonly bleed into the ventricle or into parenchyma. This probably accounts for why the occurrence of vasospasm is uncommon in AVM cases. Spontaneous hemorrhage during the perioperative period as a result of variations in systemic blood pressure is probably less likely as well, due to a “buffering” capacity of the fistula on changes in systemic pressure.
The morbidity of spontaneous AVM hemorrhage is controversial, , but estimates run from very low to as high as 25–50%. The most recent prospective, longitudinal study data suggest that earlier estimates may be overestimates, and that hemorrhage, either at initial presentation or during follow-up of untreated AVM patients, appears to carry a lower morbidity than ICH from other causes.
Consistent with the risk factors described above, the primary reason to treat a patient with an AVM is to protect him or her against future spontaneous intracranial hemorrhage, although more rarely, treatment may be undertaken for control of progressive neurological deficits or intractable seizures. Lacking any specific medical therapies for this purpose, treatment options are currently limited to three modes for treatment of AVMs: endovascular embolization, radiosurgery, and microsurgical excision. Treatment strategies, especially for complex lesions, frequently involve more than one modality. In general, endovascular therapy is performed as a preparatory adjunct to surgery. Using various glues or other embolic materials like Onyx, the blood supply to the fistula can be reduced, sometimes in several stages. This has the theoretical advantage of allowing surrounding brain regions to adapt to the circulatory changes. As a preoperative adjunct, embolization is thought to facilitate operative removal with less bleeding and seems to be associated with better surgical outcome. Embolization also can eliminate deep vascular pedicles that might be difficult to control surgically.
The risks of invasive therapy can be estimated using scales adapted for different treatment modalities, most importantly for surgery and radiotherapy. , Neurosurgeons are confident in their recommendations for microsurgical resection with most low-grade AVMs (Spetzler-Martin grade I–III), based on numerous reports detailing excellent results. However, all treatment modalities—endovascular therapy, surgical and radiosurgical—continue to be reported as carrying a substantial risk of disability. ,
For example, a meta-analysis of 2,425 patients from 25 sources undergoing invasive treatment described an aggregate mortality of 3.3% with permanent morbidity of 8.6%, ranging from 1.5% to 18.7%. Protection from spontaneous ICH by partial, non-curative endovascular embolization therapy has been suggested but does not have rigorous support. , Pre-surgical embolization, thought to enhance safety of surgical resection, has its own inherent morbidity varying from 4% to 9%. , A recent multicenter overview on endovascular AVM therapy (commissioned by the 2005 World Federation of Interventional and Therapeutic Neuroradiology meeting) revealed stable frequencies of self-reported treatment-related complications in numerous well-established international centers, in the range 9–12%, but going as high as 22%.
It is worthwhile noting that those risk factors that increase the risk of leaving the lesion untreated (i.e., increase the risk of spontaneous hemorrhage) do overlap with those characteristics that increase risk of surgical intervention, but they are not the same. The most widely used surgical risk score is the Spetzler-Martin scheme. Any deep venous drainage increases surgical risk, but only exclusively deep venous drainage appears to influence rupture risk. Larger size is an important component of increased surgical risk, but does not affect natural history risk or may even represent a protective effect owing to low intranidal pressure with high flow lesions. Eloquence, an important attribute of where the AVM is located, strongly influences surgical risk, but has no effect on natural history risk. The point here is that when one is discussing “high risk lesions,” it is important to specify whether one is talking about natural history or treatment risk.
Radiosurgical treatment offers a means to treat surgically inaccessible lesions and appears to be useful for smaller lesions. It is less efficacious for lesions > 2–3 cm. Further, patients who have received radiosurgical treatment are still exposed to the risk of new bleeding until the AVM is obliterated, usually after a course of 2–3 years, termed the latency period . During the latency period, risk for ICH may decrease in patients who presented with hemorrhage, but not in those who were unruptured at presentation. The frequency of neurological complications from radiotherapy of brain AVMs is generally similar to the complication rate from surgical and endovascular treatment. The Randomized Trial of Brain Unruptured AVMs (ARUBA) investigators performed a systematic review of the literature from 1990 or later of prospective studies with at least 30 patients, and yielded 16 studies on 3,854 patients undergoing radiosurgery and a mean rate of treatment-related permanent neurological deficits in the range of 6–7%; obliteration rates, completeness and type of follow-up varied greatly.
The most controversial aspect of treatment is offering potentially high-risk invasive therapy for those who have not yet ruptured. Patients with unruptured lesions are at the highest risk for postoperative deficits. The presenting hemorrhage may, in effect, perform some of the dissection, in that the hemorrhage cavity is an attractive approach to the lesion. Further, patients who are recovering from a hemorrhage-induced deficit may not have reached the final level of spontaneous recovery that may continue into the postoperative period, thus masking operative injury. One of the most important studies of recent times is the ARUBA trial, an international randomized controlled trial (RCT) to test whether it is best medical therapy or procedural intervention that results in superior outcomes. The mean follow-up was 33.3 months in 223 patients, including 114 assigned to interventional therapy and 109 to medical management, to compare the risk of death and symptomatic stroke. The superiority of the medical management group was demonstrated based on the incidences of strokes or death in 11 (10.1%) of the medical management group compared with 35 (30.7%) of the interventional therapy group. While the ARUBA trial suggests that medical management alone is superior for the prevention of death or stroke in patients with unruptured brain AVMs, there are ongoing debates about the applicability to all patients, given the heterogeneity of the disease and the small sample sizes, short follow-up, and poor participation of American centers in the trial.
Etiology and pathogenesis
The genesis of AVMs has been enigmatic. Unlike the association of antecedent head trauma or other injuries with the pathogenesis of dural arteriovenous fistulae (DAVF), environmental risk factors for AVMs are lacking. There is remarkably little evidence for the common assertion that AVMs are congenital lesions as a result of embryonic maldevelopment during the 4th to 8th week, considering high utilization of prenatal ultrasound (vein of Galen lesions are not true AVMs). Further, there have been multiple reports of AVMs that grow or regress, including de novo AVM formation. Inciting event(s) might include the sequelae of even relatively modest injury from an otherwise unremarkable episode of trauma, infection, inflammation, irradiation, compression, or some underlying structural defect. In susceptible individuals, one might posit some degree of localized venous hypertension from microvascular thrombosis, perhaps associated with a state of relative thrombophilia. All of these events may synergize and involve some underlying development defect that otherwise does not come to clinical attention. The scarce data available on longitudinal assessment of AVM growth suggest that approximately 50% of cases display interval growth. Consistent with growth is the many-fold higher endothelial proliferation rate in AVM surgical specimens, compared to the control brain.
Available evidence points towards an active angiogenic and inflammatory lesional phenotype rather than a static congenital anomaly. There are a host of abnormal signals present in the lesional tissue. , A prominent feature of the AVM phenotype is relative overexpression of VEGF-A, at both the mRNA and protein level. VEGF may contribute to the hemorrhagic tendency of AVMs, extrapolating from animal models. Other upstream factors that may contribute to AVM formation might include Homeobox genes, such as excess pro-angiogenic Hox D3 or deficient anti-angiogenic Hox A5. The vascular phenotype of AVM tissue may be explained, in part, by inadequate recruitment of periendothelial support structure, which is mediated by angiopoietins and Tie-2 signaling. For example, angiopoietin-2 (Ang-2), which allows loosening of cell-to-cell contacts, is overexpressed in the perivascular region in AVM vascular channels.
Vascular remodeling is facilitated by proteases and is necessary to form the enlarged vascular elements in the nidus. A key downstream consequence of VEGF and other angiogenic activity is MMP expression. MMP-9 is of particular interest and is an order of magnitude higher in AVM than control tissue. , Inflammatory markers that are overexpressed include myeloperoxidase (MPO) and IL-6, as well as higher immunoglobulin levels than control the brain, perhaps suggesting lymphocytic contributions. The presence of T-lymphocytes in BAVMs also suggest the possibility of an independent cell-mediated immunological mechanism in AVM pathogenesis.
Brain AVMs are usually sporadic, but sometimes familial. The most promising candidate genes/pathways for brain AVM pathogenesis relate to hereditary hemorrhagic telangiectasia (HHT), an autosomal dominant disorder of mucocutaneous fragility and AVMs in various organs including the brain. There are now at least five genes associated with HHT, but the two main subtypes of HHT (HHT1 and 2) are caused by loss-of-function mutations in two genes originally implicated in TGF-β signaling pathways ( Fig. 15.1 ). The first is endoglin (ENG), which codes for an accessory protein of TGF-β receptor complexes. The second is activin-like kinase 1 ( ALK1 , or ACVLR1 ), which codes for a transmembrane kinase also thought to participate in TGF-β signaling. Recent data suggest that ALK1 may also signal through BMP-9, and that ENG can potentiate the signal. ,

As a class, the inherited AVMs in HHT have some distinguishing morphological features, such as smaller size, multiplicity and more superficial location, but are generally similar to the sporadic lesions and cannot be distinguished individually on the basis of their angioarchitecture. , Together, about 10% of HHT1 and HHT2 patients display brain AVMs. This 10% figure is some 1000 times more frequent than the prevalence of brain AVM in the normal population (about 10/100,000 or .01%). Therefore, one could view frank mutations in ALK1 and ENG as “hyper-risk” factors for the brain AVM phenotype.
Such a greatly elevated risk of brain AVM development in the Mendelian disorders raises the possibility that germline sequence variants of these and other genes may likewise pose a significant risk for sporadi c brain AVM development. An intronic SNP in ALK1 has been associated with a twofold elevated risk of the developing sporadic brain AVM, a finding replicated in an independent cohort. , This SNP probably causes in-frame exon skipping, and may result in an ALK1 protein variant that lacks a transmembrane domain. An intriguing but unproven mechanism would be excess soluble receptor in the extracellular matrix that binds ligand and prevents it from normal signaling.
It is highly unlikely that a single polymorphic gene is responsible for AVM formation. Rather, it is mostly likely some combination of (a) an inciting event or developmental defect, (b) some genetic alteration in either ALK1 or ENG signaling or a closely related pathway, and (c) a set of modifier genes or conditions. For example, there are multiple genetic loci that appear to control VEGF factor-induced angiogenesis. , Such a conspiracy of factors is suggested by the observation that ENG-deficient mice spontaneously form vascular dysplasia, and that the response can be amplified using viral transduction to overexpress VEGF in the mouse brain. Recent advances in genetic mutation, in combination with angiogenic stimulation, have made it possible to establish testable adult mouse brain AVM models. Mutation of ALK1 and ENG genes in different vascular cell types can mimic many phenotypes of human brain AVM and can thus be used for studying brain AVM pathogenesis and testing new therapies.
Etiology and pathogenesis
The genesis of AVMs has been enigmatic. Unlike the association of antecedent head trauma or other injuries with the pathogenesis of dural arteriovenous fistulae (DAVF), environmental risk factors for AVMs are lacking. There is remarkably little evidence for the common assertion that AVMs are congenital lesions as a result of embryonic maldevelopment during the 4th to 8th week, considering high utilization of prenatal ultrasound (vein of Galen lesions are not true AVMs). Further, there have been multiple reports of AVMs that grow or regress, including de novo AVM formation. Inciting event(s) might include the sequelae of even relatively modest injury from an otherwise unremarkable episode of trauma, infection, inflammation, irradiation, compression, or some underlying structural defect. In susceptible individuals, one might posit some degree of localized venous hypertension from microvascular thrombosis, perhaps associated with a state of relative thrombophilia. All of these events may synergize and involve some underlying development defect that otherwise does not come to clinical attention. The scarce data available on longitudinal assessment of AVM growth suggest that approximately 50% of cases display interval growth. Consistent with growth is the many-fold higher endothelial proliferation rate in AVM surgical specimens, compared to the control brain.
Available evidence points towards an active angiogenic and inflammatory lesional phenotype rather than a static congenital anomaly. There are a host of abnormal signals present in the lesional tissue. , A prominent feature of the AVM phenotype is relative overexpression of VEGF-A, at both the mRNA and protein level. VEGF may contribute to the hemorrhagic tendency of AVMs, extrapolating from animal models. Other upstream factors that may contribute to AVM formation might include Homeobox genes, such as excess pro-angiogenic Hox D3 or deficient anti-angiogenic Hox A5. The vascular phenotype of AVM tissue may be explained, in part, by inadequate recruitment of periendothelial support structure, which is mediated by angiopoietins and Tie-2 signaling. For example, angiopoietin-2 (Ang-2), which allows loosening of cell-to-cell contacts, is overexpressed in the perivascular region in AVM vascular channels.
Vascular remodeling is facilitated by proteases and is necessary to form the enlarged vascular elements in the nidus. A key downstream consequence of VEGF and other angiogenic activity is MMP expression. MMP-9 is of particular interest and is an order of magnitude higher in AVM than control tissue. , Inflammatory markers that are overexpressed include myeloperoxidase (MPO) and IL-6, as well as higher immunoglobulin levels than control the brain, perhaps suggesting lymphocytic contributions. The presence of T-lymphocytes in BAVMs also suggest the possibility of an independent cell-mediated immunological mechanism in AVM pathogenesis.
Brain AVMs are usually sporadic, but sometimes familial. The most promising candidate genes/pathways for brain AVM pathogenesis relate to hereditary hemorrhagic telangiectasia (HHT), an autosomal dominant disorder of mucocutaneous fragility and AVMs in various organs including the brain. There are now at least five genes associated with HHT, but the two main subtypes of HHT (HHT1 and 2) are caused by loss-of-function mutations in two genes originally implicated in TGF-β signaling pathways ( Fig. 15.1 ). The first is endoglin (ENG), which codes for an accessory protein of TGF-β receptor complexes. The second is activin-like kinase 1 ( ALK1 , or ACVLR1 ), which codes for a transmembrane kinase also thought to participate in TGF-β signaling. Recent data suggest that ALK1 may also signal through BMP-9, and that ENG can potentiate the signal. ,
As a class, the inherited AVMs in HHT have some distinguishing morphological features, such as smaller size, multiplicity and more superficial location, but are generally similar to the sporadic lesions and cannot be distinguished individually on the basis of their angioarchitecture. , Together, about 10% of HHT1 and HHT2 patients display brain AVMs. This 10% figure is some 1000 times more frequent than the prevalence of brain AVM in the normal population (about 10/100,000 or .01%). Therefore, one could view frank mutations in ALK1 and ENG as “hyper-risk” factors for the brain AVM phenotype.
Such a greatly elevated risk of brain AVM development in the Mendelian disorders raises the possibility that germline sequence variants of these and other genes may likewise pose a significant risk for sporadi c brain AVM development. An intronic SNP in ALK1 has been associated with a twofold elevated risk of the developing sporadic brain AVM, a finding replicated in an independent cohort. , This SNP probably causes in-frame exon skipping, and may result in an ALK1 protein variant that lacks a transmembrane domain. An intriguing but unproven mechanism would be excess soluble receptor in the extracellular matrix that binds ligand and prevents it from normal signaling.
It is highly unlikely that a single polymorphic gene is responsible for AVM formation. Rather, it is mostly likely some combination of (a) an inciting event or developmental defect, (b) some genetic alteration in either ALK1 or ENG signaling or a closely related pathway, and (c) a set of modifier genes or conditions. For example, there are multiple genetic loci that appear to control VEGF factor-induced angiogenesis. , Such a conspiracy of factors is suggested by the observation that ENG-deficient mice spontaneously form vascular dysplasia, and that the response can be amplified using viral transduction to overexpress VEGF in the mouse brain. Recent advances in genetic mutation, in combination with angiogenic stimulation, have made it possible to establish testable adult mouse brain AVM models. Mutation of ALK1 and ENG genes in different vascular cell types can mimic many phenotypes of human brain AVM and can thus be used for studying brain AVM pathogenesis and testing new therapies.
Cerebral circulatory changes in patients with arteriovenous malformations
There are two primary characteristics of the cerebral circulatory changes brought about by AVMs. Rapid shunt flow results in an increased total amount of bulk flow through the AVMs. This increased flow results in cerebral arterial hypotension along the path of the shunt. Patients with AVMs have a progressive decrease in cerebral arterial pressure that proceeds from the circle of Willis to AVMs nidus ( Fig. 15.2 ). The corollary of this observation is that circulatory beds in parallel with the shunt system will be perfused at lower-than-normal pressures, even if flow remains relatively normal.

In patients with large, high flow AVMs, there may be normal brain regions in which cerebral arterial hypotension is below the range of normal autoregulation. Despite significant cerebral arterial hypotension, a majority of patients are free from ischemic symptoms. Hypotensive normal brain regions can be demonstrated to have normal rates of tissue perfusion, implying some adaptive change in total cerebrovascular resistance.
This phenomenon may be explained by “adaptive autoregulatory displacement.” In vascular territories adjacent to AVMs, the lower limit of the autoregulation curve appears to be shifted to the left, which is opposite of the effect of chronic systemic (essential) arterial hypertension on the cerebral autoregulation curve. This adaptive shift to the left places the lower limit at a level considerably lower than the lower limit postulated for the normal brain (50 or 60 mmHg). , Therefore, the presence of chronic hypotension does not necessarily result in vasoparalysis in the arteriolar resistance bed. There is generally a preserved responsiveness to CO 2 pre- and post-surgical resection, which lends further support to the notion of intact autoregulatory capacity.
Historically, it has been believed that it is not the AVM itself but rather decreased perfusion pressure in adjacent, functional tissue that is responsible for both pre-treatment ischemic and post-treatment hyperemic symptoms, namely “cerebral steal” and “normal perfusion pressure breakthrough” (NPPB). These widely discussed concepts have limited anecdotal evidence to support their existence.
Cerebral steal has been suggested as an explanation for focal neurologic deficits in AVM patients; “steal” is assumed to be attributable, by inference, to local hypotension and hypoperfusion. It has been postulated that arteriolar vascular resistance in territories adjacent to AVMs is at or near a state of maximal vasodilation, and, therefore, “steal” ensues if perfusion pressure decreases. Although cerebral arterial hypotension in normal brain areas is common in AVM patients, clinical presentations with focal neurological deficits are rare (10%). Moreover, there is no relation between local hypotension and focal neurologic deficits. It is likely that local mass effects from the abnormal vessels of the AVMs are more important than local “hemodynamic failure” to account for symptomatic focal neurologic deficit unrelated to intracerebral hemorrhage or seizure activity.
The intraoperative appearance of diffuse bleeding from the operative site or brain swelling, and the postoperative occurrence of hemorrhage or swelling have been attributed to NPPB or “hyperemic” complications. There are difficulties in studying NPPB-type complications. First, there appear to be very heterogeneous sets of criteria used by different authors. Second, the incidence of this type of postoperative complication is probably lower than 5%. The increase in global CBF after AVM resection appears to be associated with NPPB-type complications, but there is no relationship with preoperative regional arterial hypotension. NPPB is attributed to cerebral hyperemia due to repressurization of previously hypotensive regions. This theory assumes that the chronic dilatation of vessels in the hypotensive/ischemic territory leads to a loss of autoregulation. However, there are several observations contradicting this theory. First, cerebral hyperemia after an AVM resection is global and not limited to the ipsilateral side of the AVM. Second, there is no relationship between CBF changes after resection and the degree of arterial hypotension induced by AVM shunts. Third, in hypotensive regions, autoregulatory response is not impaired, but intact and shifted to the left. Finally, cerebrovascular reactivity to carbon dioxide is preserved after AVM resection, suggesting that the vessels in previously hypotensive territories are not “paralyzed.” All of these considerations notwithstanding, postoperative brain swelling and hemorrhage will be favored by uncontrolled systemic blood pressure. Although the mechanisms are still unclear, it seems reasonable to hypothesize that barrier integrity in the circulation is compromised by excessive protease activity or growth factor elaboration, as is probably the case in the setting of post-reperfusion hemorrhage after ischemic stroke.
The states of “steal” and “normal perfusion pressure breakthrough” probably do exist in some minority of cases, but if they exist, they are the exception rather than the rule. As regards perioperative management, the diagnosis of NPPB should be a diagnosis of exclusion after all other correctable causes for malignant brain swelling or bleeding have been excluded. In addition to other supportive and resuscitative measures, preventing postoperative hypertension may be useful in preventing and treating this syndrome.
Perioperative anesthetic management
Patients with AVMs often undergo several diagnostic (CT, MRI, PET) and therapeutic (interventional neuroradiology, radiosurgery, neurosurgery) procedures that may require anesthesia. Anesthetic management of these patients follows general goals of neuroanesthesia to maintain adequate CPP and to prevent increases in ICP, and focuses on preventing and managing serious perioperative complications, such as intracranial bleeding and NPPB. The following discussion of perioperative anesthetic management will concentrate on anesthesia for neuroradiologic and intraoperative procedures.
Preoperative Management
AVM resection is rarely emergent, unless there is a need for immediate surgical evacuation of an intracranial hematoma. Thus, a careful review of the patient’s preoperative status and assessment of potential intraoperative difficulties should be possible. Most patients present with intracranial hemorrhage, new seizures or neurological deficits. Preexisting medical conditions should be optimized. The impact of any neurologic dysfunction, possible use of anti-seizure medications, the location (eloquent areas) and size of the AVM (possibility for significant blood loss), and intraoperative neurophysiologic monitoring should be factored into the anesthetic management plan regarding the choice of monitoring, vascular access, anesthetic agents, vasoactive drugs, muscle relaxants, and perioperative airway control.
Intraoperative Management
Monitoring
In addition to routine monitors—EKG, pulse oximeter, end-tidal CO 2 and temperature—central venous catheters may be considered for use during resection of large AVMs when there is a high risk for significant intraoperative blood loss. Intra-arterial catheters are routinely used during AVM resection for continuous, direct blood pressure monitoring and arterial blood sampling. Since hypertensive episodes are not commonly associated with AVM rupture, intra-arterial catheters are often placed after induction of anesthesia. During AVM embolization, continuous intra-arterial blood pressure monitoring will help in the diagnosis and treatment of AVM rupture. Fortunately, these potentially devastating complications are rare (1–2%).
Unfortunately, our ability to routinely monitor the central nervous system lags far behind our ability to monitor other systems, and the development of suitable technologies is still in its infancy. SSEP, MEP, and EEG monitoring may be used intraoperatively, and direct cerebral blood flow monitoring has been proposed by several groups. At present, this is an institution-specific endeavor and is not routinely performed. Jugular venous saturation monitoring has been proposed as index of throttling the shunt fraction through the fistula.
Transduction of intravascular pressures in the operative field may aid the surgeon in differentiating arterial and venous structures. In certain cases, it may assist in the decision of whether a draining vein interfering with surgical access to the nidus can be sacrificed. Proximal arterial pressure is measured during a temporary occlusion of the vein; if the pressure does not change, it implies that alternate venous pathways are sufficient to prevent distention of the nidus and rupture. Technically, direct puncture of feeding arteries and draining veins in the operative field using 26-g needles is a simple procedure with minimal risk.
Vascular Access
The likelihood for significant intraoperative blood loss should be discussed preoperatively with the neurosurgeon. Multiple large-bore intravenous access for rapid blood administration and the availability of appropriate blood products are mandatory. Preoperative planning may include consideration of a central venous catheter if there is potential for significant intraoperative blood loss.
Anesthetic Technique
Choice of Agents
Scientific literature provides minimal guidance regarding anesthetic management of patients with AVMs. The choice of anesthetic agents varies widely among institutions and is made largely to follow basic neuroanesthesia principles and co-existing diseases. The most commonly used neuroanesthesia techniques at UCSF can be found on our website at neuroanesthesia.ucsf.edu .
Intracranial pressure control, which is often discussed with regards to the anesthetic care of neurosurgical patients, is usually not an issue with the AVM patient coming for elective resection, as the lesion does not displace, but rather replaces normal brain structures. Nonetheless, these patients may have decreased intracranial compliance, especially if significant venous hypertension is present, so the usual caveat about avoiding an anesthetic technique that results in significant cerebral vasodilation is a reasonable choice.
Patients having their AVM embolized at our institution routinely receive a general anesthetic. During embolization procedures, the anesthetic goals are to maintain an immobile patient with adequate CPP and to plan for immediate post-procedure neurologic examination. In addition to these goals, during AVM resection, the anesthetic technique should minimize brain volume in order to reduce retractor-induced cerebral ischemia. For both procedures, there should also be a plan in place for the treatment of potential complications, such as intracerebral hemorrhage during embolization and significant bleeding and/or brain swelling during and after surgery (see below).
Neurologically intact patients may be premedicated with benzodiazepines (midazolam). Propofol, thiopental or etomidate can be used to induce anesthesia, propofol being most commonly used. Maintenance of anesthesia can be achieved with varying combinations of propofol/narcotic/inhalation anesthetic. Total intravenous anesthetic techniques or combinations of inhalational and intravenous methods may optimize rapid emergence from anesthesia and allow for SSEP and MEP monitoring. There is no evidence that the choice of an inhalation anesthetic, narcotic or muscle relaxant has an intrinsic, independent effect on outcome.
Some centers traditionally use barbiturate loading during the resection of AVM for metabolic suppression to afford additional protection against cerebral ischemia, resulting in, perhaps, a greater degree of brain relaxation and protection against acute hyperemia. Barbiturates can be titrated to an EEG-endpoint of burst-suppression. The main price to be paid for barbiturate use is delayed emergence and forgoing an early postoperative neurologic exam. There is no evidence that outcome is affected. Other intravenous anesthetic agents, such as propofol and etomidate, can also be titrated to the effect of burst suppression. ,
Nonpharmacologic Cerebral Protection
The goals of modern neuroanesthesia should optimize brain protective therapy. There are a number of basic considerations that will maximize nonpharmacologic cerebral protection (summarized in Table 15.1 ). There are two general types of damage towards which protective efforts are guided: neurosurgical (anatomic) and anesthetic (physiologic) trespass. Possible mechanisms of injury from the neurosurgeon include brain retraction, direct vascular injury (ischemia, thrombosis, venous occlusion), and mechanical disruption of neuronal tissue or white matter tracts. Anesthetic injury may result from systemic hypo- or hypertension, decreased O 2 content, hypo-osmolarity or hyperglycemia. It must be stressed that mechanisms of damage are interactive. For example, modest amounts of brain retraction coupled with a modest reduction of systemic blood pressure may have synergistic effects on neurologic outcome.
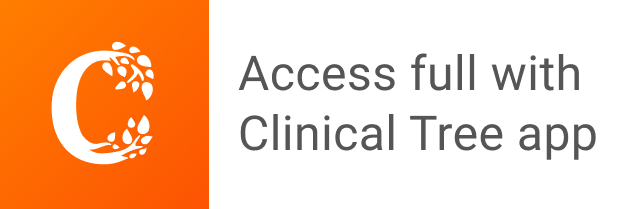