where y is the variable of interest (e.g., basal metabolic rate), a is the allometric coefficient, and PWR is the allometric exponent. The value of the PWR has been the subject of much debate. Basal metabolic rate (BMR) is the most common physiological variable investigated, although camps are divided over its PWR value, 2/3 (i.e., body surface area) or ¾.
In all species including humans, the log of basal metabolic rate (BMR) plotted against the log of body weight produces a straight line with a slope of ¾ (Fig. 3.1). Fractal geometry is used to mathematically explain this phenomenon [6, 7].


Fig. 3.1
A comparison of the temperature- standardized relation for whole-organism metabolic rate as a function of body mass. The “allometric ¾ power model” fits for unicells, poikilotherms, and homeotherms, uncorrected for temperature, are also shown. From Gillooly JF et al. Effects of size and temperature on metabolic rate. Science 2001; 293: 2248–2251, with permission
The ¾ power law for metabolic rates was derived from a general model that describes how essential materials are transported through space-filled fractal networks of branching tubes. A great many physiological, structural, and time-related variables scale predictably within and between species with weight (W) exponents (PWR) of ¾, 1, and ¼, respectively [8]. These exponents have applicability to pharmacokinetic parameters such as clearance (CL), volume (V) and half-time [8]. The factor for size (Fsize) for total drug clearance, standardized to a 70 kg person, may be expected to scale with a power of ¾:


Maturation
Allometry alone is insufficient to predict the clearance of drugs in neonates and infants from adult estimates [9–11]. The addition of a model describing maturation is required. The sigmoid hyperbolic or Hill model [12] is useful for describing this maturation process (MF):
The TM50 describes the maturation half-time, and the Hill coefficient relates to the slope of this maturation profile. It is possible that there is asymmetry about the point of inflecion and the addition of an extra parameter describing this asymmetry can be used to provide extra flexibility for this empirical function [13].

Maturation of clearance begins before birth, suggesting that PMA would be a better predictor of drug elimination than postnatal age (PNA). The fetus is capable of metabolizing drugs. There are distinct patterns associated with isoform-specific developmental expression of the cytochrome P450 (CYP) enzymes. For example, CYP2D6 has been detected in preterm neonates as young as 25 weeks’ PMA [14].
Organ Function
Changes associated with normal growth and development can be distinguished from pathological changes in organ function (OF) [5]. Pharmacokinetic parameters (P) can be described in an individual as the product of size (Fsize), maturation (MF) and organ function (OF) influences where Pstd is the value in a standard size adult without pathological changes in organ function [5].
Organ function is typically decreased in the presence of disease. However, it may also be increased by drugs. Phenobarbitone, a drug commonly given to neonates with seizures, can induce enzyme activity of a number of enzyme systems responsible for clearance [15] such as CYP1A2, CYP2C9, CYP2C19, CYP3A4, and UDP-glucuronosyltransferase (UGT) [16–18]. Phenobarbitone can increase bilirubin clearance in neonates through UGT induction [15]. Although an effect on the response to ketamine has been demonstrated in older children [19], there are no comparable examples in neonates to date.

Neonatal Pharmacokinetic Differences
Absorption
Anesthetic drugs are primarily administered via the intravenous and inhalational routes, although premedication and postoperative pain relief may be administered enterally. Drug absorption after oral administration is slower in neonates than in children due to delayed gastric emptying (Fig. 3.2). Adult absorption rates may not be reached until 6–8 months of PNA [20–22]. Congenital malformations (e.g., duodenal atresia), coadministration of drugs (e.g., opioids) or disease characteristics (e.g., necrotizing enterocolitis) may further affect the variability in absorption. Delayed gastric emptying and reduced clearance may dictate reduced doses and frequency of repeated drug administration. For example, a mean steady state target paracetamol concentration greater than 10 mg/L at trough can be achieved by an oral dose of 25 mg/kg/day in preterm neonates at 30 weeks, 45 mg/kg/day at 34 weeks and 60 mg/kg/day at 40 weeks’ PMA [23]. Because gastric emptying is slow in preterm neonates, dosing may only be required twice a day [23]. In contrast, the rectal administration of some drugs (e.g., thiopental, methohexital) is more rapid in neonates than adults undergoing cardiac catheter study or radiological sedation. However, the interindividual absorption and relative bioavailability variability may be more extensive compared to oral administration, making rectal administration less suitable for repeated administration [24].


Fig. 3.2
Simulated mean predicted time-concentration profiles for a term neonate, a 1-year-old, infant and a 5-year-old child given paracetamol elixir. The time to peak concentration is delayed in neonates due to slow gastric emptying and reduced clearance (from Anderson BJ et al. Anesthesiology 2002; 96:1336–45)
The larger relative skin surface area, increased cutaneous perfusion and thinner stratum corneum in neonates increase systemic exposure of topical drugs (e.g., corticosteroids, local anesthetic creams, antiseptics). Neonates have a greater tendency to form methemoglobin because of reduced methemoglobin reductase activity compared with older children. Furthermore, fetal hemoglobin is more readily oxidized compared with adult hemoglobin. Combined with an increased transcutaneous absorption, these have resulted in reluctance to apply repeat topical local anesthetics such as EMLA® (lidocaine-prilocaine) cream in this age group [25]. Similarly, cutaneous application of iodine antiseptics in neonates may result in transient hypothyroidism.
Inhalational anesthetic delivery is determined largely by alveolar ventilation and functional residual capacity (FRC). Neonates have increased alveolar ventilation to FRC ratio compared with adults, primarily the result of an increased metabolic demand for oxygen, which drives an increase in alveolar ventilation. Consequently, the alveolar to inspired fractions and therefore the blood to inspired partial pressure of anesthetics reach equilibration more rapidly in neonates than in children and adults [26]. The greater cardiac output and greater fraction of the cardiac output distributed to vessel-rich tissues (i.e., a clearance factor) and the lower tissue/blood solubilities (i.e., a volume factor) further contribute to the more rapid washin of inhalational anesthetics in early life [27, 28].
Disease characteristics may also contribute to the variability in the absorption of inhaled anesthetics. Right-to-left shunts affect the washin of inhalational anesthetics to a greater extent than left-to-right shunts. Induction of anesthesia may be slowed by right-to-left shunting of blood in neonates suffering cyanotic congenital cardiac disease or intrapulmonary (right-to-left) conditions. This slowing is greater with the less soluble anesthetics (e.g., nitrous oxide, sevoflurane) than the more soluble ones (e.g., halothane). Left-to-right shunts usually have minimal impact on uptake because cardiac output is increased so that systemic tissue perfusion is maintained at normal levels.
Distribution
Distribution describes the movement from systemic circulation into various body compartments, tissues, and cells. Distribution is influenced by body composition, protein binding, hemodynamics (e.g., regional blood flow) and membrane permeability. Disease processes may also impact the distribution of drugs.
Body Composition
Total body water and extracellular fluid (ECF) [29] decrease throughout gestation, the neonatal period, and childhood (Fig. 3.3), whereas the percent of body weight contributed by fat increases from 3 % in a 1.5-kg preterm neonate to 12 % in a full-term neonate and then doubles by 4–5 months of postnatal age. These changes in the body composition substantively affect the volumes of distribution of drugs. Polar drugs such as depolarizing and non-depolarizing neuromuscular-blocking drugs (NMBDs) distribute rapidly into the ECF, but enter cells more slowly. The initial dose of such drugs is consequently greater in the neonate than in the child or adult. Lipid-soluble drugs may also have a larger volume of distribution in neonates. The volume of distribution at steady state of fentanyl is 5.9 (SD 1.5) L/kg in a neonate compared with 1.6 (SD 0.3) L/kg in an adult [30]. This may explain the reduced respiratory depression after doses as large as 10 μg/kg in full-term neonates. However, high-dose therapy (50 μg/kg) results in a prolonged effect in neonates due to reduced clearance. In the case of propofol, reduction in the plasma concentration after induction of anesthesia is attributable to redistribution rather than rapid clearance. Neonates have less body fat and muscle content than older children. Hence, less propofol is apportioned to these “deep” compartments, attenuating the redistribution of propofol. If repeat doses of propofol are administered, they may accumulate in the blood and brain and delay recovery.


Fig. 3.3
Body water compartment changes during growth (used with permission from Friis-Hansen B. Changes in body water compartments during growth. In: Linneweh F, editor. Die Physiologische Entwicklung des Kindes. Berlin, Germany. Springer-Verlag. 1959)
Plasma Proteins
The plasma alpha 1-acid glycoprotein (AAG) and albumin concentrations in neonates are reduced, albeit with a broad range of scatter (e.g., AAG 0.32–0.92 g/L), but reach adult concentrations by 6 months of postnatal age [31–33]. AAG is an acute phase reactant that increases after surgical stress. This increases the total plasma concentrations for low to intermediate extraction drugs such as bupivacaine that bind to AAG [34].
The concentration of unbound bupivacaine, however, will not change because the clearance of unbound bupivacaine depends only on the intrinsic metabolizing capacity of the liver. Any increase in unbound concentrations, for example, during long-term epidural infusions, is attributable to a reduced clearance rather than a decrease in the AAG concentration [35]. Total bupivacaine concentrations increase in the first 24 h after surgery in neonates who are receiving a continuous epidural infusion of bupivacaine; unbound bupivacaine concentrations, however, have not been studied. This increase in total bupivacaine is attributable in part to an increase of AAG. This increase in total bupivacaine, combined with reports of seizures in infants given epidural bupivacaine infusion, has led to recommendations to stop epidural infusions at 24 h. However, it is the unbound bupivacaine concentration that is responsible for the CNS effects, and this is determined by the clearance of the unbound bupivacaine. Clearance, the pivotal variable in the elimination of unbound bupivacaine, is reduced in neonates. In addition, clearance shows large interindividual variability, which means that unbound bupivacaine concentrations may increase steadily in some individuals with very low clearance. The lack of knowledge regarding the clearance of bupivacaine in each neonate precludes pronouncing the duration of a safe bupivacaine infusion for all [36].
Plasma albumin concentrations are least in preterm neonates but increase steadily, approximating adult values by 5 months of postnatal age. Binding capacity approaches adult values by 1 year of age. In addition, free fatty acids and unconjugated bilirubin compete with acidic drugs (e.g., ibuprofen, ceftriaxone) for albumin binding. The induction dose of thiopental in neonates is less than it is in children. It is possible that this is related to the decreased binding of thiopental to plasma albumin; 13 % of the drug is unbound in neonates compared with 7 % in adults [37].
Regional Blood Flows
The initial phase of distribution reflects the magnitude of regional blood flow. Consequently, the brain, heart, and liver, which receive the largest fraction of cardiac output, are first exposed. Drugs are then redistributed to other relatively well-perfused tissues, such as the skeletal muscle. There is a much slower tertiary distribution to relatively under-perfused tissues of the body that is noted with long-term drug infusions. In addition to perinatal circulatory changes (e.g., ductus venosus, ductus arteriosus), there are maturational differences in relative organ mass and regional blood flow, while a symptomatic patent ductus arteriosus may also result in differences in distribution. Blood flow as a fraction of the cardiac output, to the kidney and brain, increases with age, whereas blood flow to the liver decreases through the neonatal period [38]. Cerebral and hepatic mass as proportions of body weight in the infant are much greater than in the adult [39]. While onset times are generally faster for neonates than adults (a size effect), reduced cardiac output and cerebral perfusion in neonates mean that the expected onset time after an intravenous induction is slower in neonates, although reduced protein binding may counter this observation for some drugs. Offset time is also delayed because redistribution to well-perfused and deep under-perfused tissues is more limited.
Blood-Brain Barrier
The blood-brain barrier (BBB) is a network of tight junctions that restricts paracellular diffusion of compounds between the blood and brain. Confusion over the importance of this barrier in the neonate exists, partly because of early studies on respiratory depression caused by morphine and meperidine [40]. Early investigations found that the respiratory depression after morphine was greater than that after meperidine. This difference was attributed to greater brain concentrations of morphine because of the poorly developed BBB in the neonate [40]. It was postulated that BBB permeability to water-soluble drugs, such as morphine, changes with maturation [40]. However, the neonatal respiratory depression observed after morphine could have been explained by pharmacokinetic age-related changes. For example, the volume of distribution of morphine in term neonates 1–4 days (1.3 L/kg) is reduced compared with that in infants 8–60 days of age (1.8 L/kg) and in adults (2.8 L/kg) [41]. Consequently, we might expect greater initial concentrations of morphine in neonates than in adults, resulting in more pronounced respiratory depression in the former. Respiratory depression, measured by carbon dioxide response curves or by arterial oxygen tension, is similar from 2 to 570 days of age at the same morphine blood concentration [42]. The BBB theory in this particular circumstance lacks strong evidence. It is more likely that the increased neonatal respiratory depression after morphine is due to pharmacokinetic age-related changes.
The BBB may have impact, however, in other ways. Small molecules are thought to access fetal and neonatal brains more readily than in adults [43]. BBB function improves gradually, possibly reaching maturity by full-term age [43]. Kernicterus, for example, is more common in preterm neonates than in full-term neonates. In contrast to drugs bound to plasma proteins, unbound lipophilic drugs passively diffuse across the BBB equilibrating very quickly. This may contribute to bupivacaine’s propensity for seizures in neonates. Decreased protein binding, as in the neonate, results in a greater proportion of unbound drug that is available for passive diffusion.
In addition to passive diffusion, there are specific transport systems that mediate active transport. Pathological CNS conditions can cause BBB breakdown and alter these transport systems. Fentanyl is actively transported across the BBB by a saturable ATP-dependent process, whereas ATP-binding cassette proteins such as P-glycoprotein actively pump out opioids such as fentanyl and morphine [44]. P-Glycoprotein modulation significantly influences opioid brain distribution and onset time, magnitude and duration of analgesic response [45]. Modulation may occur during disease processes, fever, or in the presence of other drugs (e.g., verapamil, magnesium) [44]. Recent evidence identified P-glycoprotein in the brain of fetuses as early as 22 weeks’ gestation [46]. The prevalence and concentration of P-glycoprotein increased with fetal maturation. Genetic polymorphisms that affect P-glycoprotein-related genes may explain differences in CNS-active drug sensitivity [47, 48].
Elimination
The main routes by which drugs and their metabolites are eliminated from the body are the hepatobiliary system, the kidneys and the lungs. The liver is the primary organ for clearance of most drugs, although the lungs have a major role for anesthetic vapors. Drug-metabolizing enzymes are generally divided into phase I and phase II reactions. Phase I reactions are non-synthetic reactions like oxidation, reduction and hydrolysis. The most important group of enzymes involved in phase I processes are the cytochrome P450 (CYP) isoenzymes. Phase II reactions convert lipid-soluble drugs to water-soluble compounds, e.g., uridine diphosphate-glucuronosyltransferase (UGT). Metabolism may result in transformation to an active drug (e.g., codeine to morphine by CYP2D6, propacetamol to paracetamol by esterase, morphine to morphine-6-glucuronide by UGT2B7) or into a toxic compound (halothane to trifluoroacetyl chloride by CYP2E1 causing halothane hepatitis).
Hepatic Metabolic Clearance
Constitutional, environmental and genetic factors all contribute to the variability in clearance, but in the young neonate, age is the dominant covariate. Most CYP isoenzymes have small phenotypic activity until birth except for CYP3A7 [49, 50]. CYP3A7 expresses its greatest activity during gestation after which its activity steadily decreases until there is no activity by 2 years [51, 52]. CYP2E1 activity surges after birth [53], CYP2D6 becomes detectable soon thereafter reaching adult levels by 2 weeks of age, and CYP3A4 and CYP2C (Fig. 3.4) are detectable during the first week, whereas CYP1A2 is the last to appear [52, 54]. Neonates depend on the immature CYP3A4 for levobupivacaine or midazolam clearance and on CYP1A2 for ropivacaine clearance, dictating reduced epidural infusion rates in this age group [1, 2, 55, 56].


Fig. 3.4
Developmental expression of human hepatic CYP2C9 enzyme (used with permission from Koukouritaki et al. [50])
CYP1A2 is 4–5 % in the neonate reaching 50 % of adult activity levels by 1 year of age [22]. Formation of the M1 metabolite of tramadol, a reflection of CYP2D6 activity [14], appears rapidly at term and reaches 84 % of mature values by 44 weeks’ PMA.
Pharmacogenomics (PG) investigates variations of DNA and RNA characteristics related to drug response that incorporates both PK and PD. Large interindividual PK variability depends to a large extent on polymorphisms of the genes that encode for metabolic enzymes [57, 58].
The effect of genetic variability on plasma cholinesterase activity and its effect on the termination of action of succinylcholine is a well-known example [59]. Another example is the CYP2D6 single nuclear polymorphism (SNP), inherited as an autosomal recessive trait that may result in poor analgesia from codeine because the active metabolite morphine is not formed [58, 60]. Both PMA and CYP2D6 activity score explained the interindividual variability in tramadol metabolism (Fig. 3.5) [51, 60]. The interplay between maturation of tramadol clearance, M1 metabolite formation and maturing GFR on M1 concentration (and subsequent analgesia) is shown in Fig. 3.6 [48]. This observation illustrates the potential relevance of polymorphisms in neonatal pharmacology.



Fig. 3.5
Tramadol M1 metabolite formation clearance (CYP2D6) increases with postmenstrual age. Rate of increase varies with genotype expression. Adopted from Allegaert [14]. Allegaert K, van den Anker JN, de Hoon JN, et al. Covariates of tramadol disposition in the first months of life. Br J Anaesth 2008;100:525–32

Fig. 3.6
Time-concentration profiles for tramadol and the M1 metabolite in neonates. CYP2D6 activity has been assigned a score of 0–3. Clearance of the parent drug is reduced in the 34-week PMA neonate compared with the 46-week PMA neonate and CYP activity has little impact on profiles. At 46 weeks both total clearance and CYP2D6 activity have increased, resulting in distinct profiles. The M1 metabolite is cleared by renal function, and the rapid maturation of glomerular filtration rate around 40 weeks’ PMA has impact on the M1 metabolite profile, resulting in a peak concentration and subsequent decrease (adapted from Allegaert et al. [14])
Some phase II isoenyzmes are mature in full-term neonates at birth (sulfate conjugation), whereas others are not (acetylation, glycination, glucuronidation) [52, 61]. Glucuronidation is important in the metabolic clearance of drugs (paracetamol, morphine, propofol) frequently administered by anesthesiologists. In the neonate, glucuronidation accounts for only 25 % of the phase II conjugation of acetaminophen, whereas in adults it accounts for ~75 % [22]. Allometric body-size scaling complemented by maturation models [8, 62] has been used to unravel the effects of maturation of the pharmacology of morphine [63–65] and paracetamol [66, 67]. Both drugs are cleared by specific isoforms (UGT1A6 and UGT2B7) [52]. In both instances, clearance is immature in the preterm 24-week PMA neonate and matures to reach adult rates by the end of the first year of life (Fig. 3.7). Dexmedetomidine is also cleared predominantly by the UGT system (UGT1A4 and UGT2B10) and has a similar maturation profile [68].


Fig. 3.7
Clearance maturation, expressed as a percentage of mature clearance, of drugs where glucuronide conjugation (paracetamol, morphine, dexmedetomidine) plays a major role. These profiles are closely aligned with glomerular filtration rate (GFR). In contrast, cytochrome P450 isoenzymes also contribute to propofol metabolism and cause a faster maturation profile than expected from glucuronide conjugation alone. Tramadol clearance maturation (phase I, CYP2D6, CYP3A) is also rapid. Maturation parameter estimates were taken from references [14, 56, 62, 64, 68, 84, 118]
Glucuronidation is the major metabolic pathway for propofol. This pathway is immature in neonates, although multiple CYP isoenzymes (CYP2B6, CYP2C9, CYP2A6) also contribute to its metabolism and cause a more rapid maturation profile than expected from glucuronidation alone [69] (Fig. 3.7). Urine collections after intravenous bolus of propofol in neonates (PNA 11 days, PMA 38 weeks) support this contention. Urinary metabolites included both propofol glucuronide and 1- and 4-quinol glucuronide in a ratio of 1:2. Hydroxylation to quinol metabolites was active in these neonates [70], contributing to the rapid increase in clearance at this age that is faster than that reported for glucuronide conjugation alone (e.g., paracetamol, morphine).
Disease characteristics also contribute to the variability in UGT-related clearance. Maturation of morphine clearance occurs more quickly in infants undergoing noncardiac surgery compared with those after cardiac surgery [71]. Neonates requiring extracorporeal membrane oxygenation [72] or positive pressure ventilation [64] also have reduced clearance. Similarly, the clearance of propofol is reduced after cardiac surgery in children [73].
Extrahepatic Routes of Metabolic Clearance
Many drugs undergo metabolic clearance at extrahepatic sites. Remifentanil and atracurium are degraded by nonspecific esterases in tissues and erythrocytes, and these processes appear mature at birth, even in preterm neonates [74]. Clearance, expressed per kilogram, is increased in younger children [75–79], likely attributable to size because clearance is similar when scaled to a 70-kg person using allometry [75]. Ester local anesthetics are metabolized by plasma butyryl-cholinesterase, which is thought to be reduced in neonates. The in vitro plasma half-life of 2-chloroprocaine in umbilical cord blood is twice that in maternal blood [80], but there are no in vivo studies of the effects of age on its metabolism. Succinylcholine clearance is increased in neonates [81, 82], suggesting butyryl-cholinesterase activity is mature at birth.
Pulmonary Elimination
The factors that determine anesthetic absorption through the lung (alveolar ventilation, FRC, cardiac output, solubility) also contribute to elimination kinetics. We might anticipate more rapid washout in neonates for any given duration of anesthesia because of the greater alveolar ventilation to FRC ratio, greater fraction of cardiac output perfusing vessel-rich tissues, reduced solubility in blood and tissues, and a reduced distribution to fat and muscle. Furthermore, younger age (as in the neonate) will speed the elimination of more soluble anesthetics such as halothane to a greater extent than the less soluble anesthetics, desflurane and sevoflurane. Halothane, and to a far lesser extent isoflurane (1.5 %) and sevoflurane (5 %), undergoes hepatic metabolism. Halothane is reported to undergo as much as 20–25 % metabolism, but at typical anesthetizing concentrations, hepatic halothane removal is extremely small [83].
Renal Elimination
Renal elimination of drugs and their metabolites occurs primarily by two processes: glomerular filtration and tubular secretion. Glomerular filtration rate (GFR) is only 10 % that of mature value at 25 weeks, 35 % at term and 90 % of the adult GFR at 1 year of age [22, 84]. Aminoglycosides are almost exclusively cleared by renal elimination and maintenance dose is predicted by PMA because it predicts the time course of renal maturation [85]. The kidney is also capable of metabolizing drugs; CYP2E1, which metabolizes ether anesthetics, is active in the kidney. The very presence of CYP2E1 in the kidney has been held responsible for the degradation of ether anesthetics and the release of nephrotoxic fluoride [86].
Immaturity of the clearance pathways can be used to our advantage when managing apnea after anesthesia in the preterm neonate. N7-Methylation of theophylline to produce caffeine is well developed in the neonate, whereas oxidative demethylation (CYP1A2) responsible for caffeine metabolism is deficient. Theophylline is effective for the management of postoperative apnea in the preterm neonate, in part because it is a prodrug of caffeine, which is effective in controlling apnea in this age group and can only be cleared slowly by the immature kidney [87].
Milrinone, an inodilator, is used increasingly in children after congenital cardiac surgery. Renal clearance is the primary route of elimination. A clearance of 9 L/h/70 kg is reported in adults with congestive heart failure, and we might anticipate that clearance in preterm neonates is reduced to 10 % of this rate because renal function is correspondingly immature in this cohort. This has been confirmed in 26-week PMA preterm infants whose milrinone clearance was 0.96 L/h/70 kg [88]. Similarly the clearance of the neuromuscular-blocking drug (NMBD) d-tubocurare can be directly correlated with GFR [89]. Some drugs such as the nonsteroidal anti-inflammatory drugs (NSAIDs) may compromise renal clearance in early life: ibuprofen reduces GFR by 20 % in preterm neonates, independent of gestational age [90, 91].
Neonatal Pharmacodynamic Differences
Children’s responses to drugs have much in common with the responses in adults once developmental PK aspects are considered [92]. The perception that drug effects differ in children arises because these drugs have not been adequately studied in pediatric populations who have size- and age-related effects as well as different diseases. Neonates, however, often have altered pharmacodynamics as well.
The minimum alveolar concentration (MAC) is commonly used to express anesthetic vapor potency. The MAC values for most vapors in neonates are less than those in infants (Fig. 3.8) [27]. The MAC of isoflurane in preterm neonates <32 weeks’ gestation was 1.28 %(SD 0.17), and in preterm neonates 32–37 weeks’ gestation was 1.41 % (SD 0.18) which in turn is less than in full-term neonates [93].


Fig. 3.8
Effect of age on the minimum alveolar concentration (MAC) of isoflurane. MAC increases as age decreases reaching a zenith in infants 1–6 months of age and decreases thereafter as age decreases to 24 weeks’ gestation [93]
Similarly, the MAC of halothane in full-term neonates (0.87 %) is less than that in infants (1.20 %), but the decrease in blood pressure and the incidence of hypotension in neonates and infants at approximately 1 MAC of halothane are similar [94].
Changes in regional blood flow may influence the amount of drug that reaches the brain. Inhalational anesthetics are thought to act via gamma-aminobutyric acid (GABAA) receptors, and receptor numbers or developmental shifts in the regulation of chloride transporters in the brain may change with age, altering the response to these anesthetics. Midazolam acts on the same receptors. Data from rodents from immediate neonates to PNA 40 days have shown developmental PD changes for sedation that mimic those observed in human childhood [95]. Such models offer potential to improve our understanding of developmental pharmacology [96].
Neonates demonstrate an increased sensitivity to the effects of neuromuscular-blocking drugs [89]. The reason for this sensitivity is unknown, but the finding is consistent with the observation that there is a threefold reduction in the release of acetylcholine from the infant rat phrenic nerve compared with the adult nerve [97, 98]. Reduced clearance and increased sensitivity prolong the duration of neuromuscular effect.
The duration of regional block with amide local anesthetic agents in infants is reduced compared with older children. Moreover, infants require a larger weight-scaled dose to achieve a similar dermatomal level when local anesthetics are given by subarachnoid block. This may, in part, be due to reduced myelination in infants, greater cerebrospinal fluid volume, increased spacing of nodes of Ranvier and the length of nerve exposed.
There is an age-dependent expression of intestinal motilin receptors and the modulation of antral contractions in neonates. Prokinetic agents may not be useful in very preterm neonates, useful only, in part, in older preterm infants but very useful in full-term infants. Similarly, bronchodilators are ineffective in neonates because of the paucity of bronchial smooth muscle that can cause bronchospasm.
Cardiac calcium stores in the endoplasmic reticulum are reduced in the neonatal heart because of immaturity. Exogenous calcium has a greater impact on contractility in this age group than in older children or adults. Conversely, calcium channel blocking drugs (e.g., verapamil) can cause life-threatening bradycardia and hypotension [99]. Catecholamine release and response to vasoactive drugs vary with age. These pharmacodynamic differences are based in part upon developmental changes in myocardial structure, cardiac innervation and adrenergic receptor function. For example, the immature myocardium has fewer contractile elements and therefore a decreased ability to increase contractility; it also responds poorly to standard techniques of manipulating preload [100]. Dopaminergic receptors are present in the pulmonary vasculature and are believed to be responsible for pulmonary vasoconstriction in preterm neonates. However, systemic vasoconstriction is greater than that observed in the pulmonary circulation, and this differential response contributes to the use of dopamine in neonates with known pulmonary hypertension after cardiac surgery. Neonates have underdeveloped sympathetic innervation and reduced stores of norepinephrine. Signs of cardiovascular α-receptor stimulation may occur at lower doses than β-receptor stimulation because β-receptor maturation lags behind α-receptor maturation during the development of the adrenergic system [101]. The preterm neonate has immature metabolic and elimination pathways leading to increased dopamine concentrations after prolonged infusions [101–104]. These maturational changes in PK and PD may contribute to dopamine’s continued popularity in the neonatal nursery while its popularity wanes in the adult population.
Pharmacodynamic Measures
In general, outcome measures are more difficult to assess in neonates than in children or adults. The common effects measured in anesthesia are circulatory and respiratory depression, neuromuscular blockade, depth of anesthesia, and sedation or pain. Circulatory responses may be assessed using heart rate and blood pressure, although more detailed analyses require echocardiogram and electrophysiology of the conduction systems. Similarly, respiratory responses may be assessed in terms of respiratory rate and gas exchange (oximetry and capnometry), but more sophisticated effects require CO2 response curves and compliance changes. Electromyography response of the adductor pollicis is a consistent effect measure for investigation of neuromuscular blockade in both neonates and adults. Differences are minor, e.g., neonates do not tolerate repetitive stimulations for as long as older children, because the acetylcholine reserves in neonates are limited. In the cases of depth of anesthesia, sedation and pain in neonates, assessing outcome variables becomes more difficult.
A common metric to assess the depth of anesthesia is the electroencephalogram (EEG) or a modification of detected EEG signals (spectral edge frequency, bispectral index, entropy). Physiological studies in adults and children indicate that EEG-derived anesthesia depth monitors provide an imprecise and drug-dependent measure of arousal. They can be used as guides for anesthesia and have improved outcomes in adults. In older children, the physiology, anatomy and clinical observations indicate the performance of the monitors may be similar to that in adults. However, ketamine provides inconsistent estimates of depth of anesthesia with the BIS. In the case of sevoflurane, the BIS in children paradoxically increases at end-tidal concentrations >3 % [105]. In infants the use of these monitors cannot yet be supported in theory or in practice [106, 107]. Both outside and during anesthesia, the EEG in infants is fundamentally different from the EEG in older children; there remains a need for specific neonate-derived algorithms if EEG-derived anesthesia depth monitors are to be used in neonates [108, 109].
The existence of an extensive number of sedation or pain scales does not mean that all assessment-related problems have been solved. Most scores are validated for the acute, procedural setting and perform less reliably for subacute or chronic pain or stress. Scoring systems seldom take into account the limited capacity of the more immature infants to mount a consistent behavioral and physiological response to pain. Validation is based on assessment of intra- and interindividual variability and correlations with neuroendocrine markers of stress or pain. Future research may provide us with objective tools to quantify pain and sedation but will have to take the maturational aspects of the neonate into account.
Induction Agents
Intravenous induction agents exert their anesthetic effects by achieving adequate cerebral concentrations to prevent undesired responses such as movement. Termination of these effects is attributed to redistribution of the drugs away from the brain rather than rapid clearance from the body, an effect that may be delayed in neonates. With less body fat and muscle in neonates, less drug is apportioned to these “deep” compartments. Delayed awakening after intravenous agents occurs in neonates because the concentration of these agents in the brain remains greater than that observed in older children as a consequence of slower redistribution.
Propofol
Mechanism
Pharmacodynamics
Integrated PK–PD studies in neonates are lacking, partly due to a lack of consistent effect measures. Consequently, the target concentration for anesthesia in neonates is unknown. The equilibration half-time (T 1/2keo) for the effect compartment is unknown but is assumed to be smaller [109] than the 3 min described in adults [112, 113]. Reduced numbers of GABAA receptors in the neonatal brain may contribute to a reduced target concentration to effect a response, but this hypothesis remains untested. A circadian night rhythm effect has been noted in an investigation of infant propofol sedation after major craniofacial surgery [114], but such an effect is unlikely in neonates who do not have established day/night sleep cycles.
Pharmacokinetics
Propofol is metabolized in the liver with an extraction ratio of approximately 0.9. Clearance is limited by the hepatic blood flow and is thus reduced in children in low cardiac output states [73]. Clearance is affected primarily by UGT1A9 (glucuronidation), with contributions from CYP2B6, CYP2C9, and CYP2A6 isoenzymes, resulting in a more rapid maturation profile than expected from glucuronide conjugation alone (Fig. 3.7).
Although propofol is widely used for target-controlled infusion (TCI) anesthesia in children, commonly used TCI data sets [113, 115–117], have only investigated propofol PK in children beyond infancy. In an effort to link neonatal data with those from children [118], Allegaert used allometry and the Hill equation [62] to suggest a maturation half-time of 44 weeks and a Hill coefficient of 4.9 [119]. Clearance at 28 weeks’ gestation is only 10 % that of the mature value and by full term reaches 38 %. Clearance in the full-term neonate achieves 90 % of the adult value (1.83 L/min/70 kg) by 30 weeks’ PMA. While postmenstrual age (PMA) is the major descriptor of maturation, it is possible that postnatal age (PNA) may also have an additional effect on maturation of propofol clearance above that predicted by PMA [69]. Further longitudinal data that examine individual neonates as they grow are required to clarify this aspect of maturation.
Adverse Effects
Propofol is used for intubation by neonatologists [120, 121] and anesthesiologists [122, 123]. While doses of 2–3 mg/kg are reported [123–125], caution is advocated in early postnatal life where a transient return to fetal circulation is possible (“flip-flop” phenomenon) due to reduced systemic vascular resistance concomitant with increased pulmonary resistance (associated with hypoxemia and acidosis) [126, 127]. Profound low cardiac output state, together with profound oxygen desaturation that was refractory to usual resuscitation measures including most inotropes, has been reported [125, 128, 129]. Additionally, hypotension of 30-min duration has been reported in preterm neonates given propofol 3 mg/kg for procedural sedation in a neonatal intensive care unit [130], although the severity of the hypotension was similar to that reported after volatile anesthetics at 1 MAC [118], Whether these episodes are attributable to hypovolemia or persistent fetal circulation is not clear, although one study was undertaken in preterm infants within a few hours after birth [125]. In other reports where propofol was administered to older preterm infants, hypotension was not detected [121, 131]. We recommend loading these infants with 10–20 mL/kg balanced salt solution before administration of propofol and atropine 0.02 mg/kg IV and preoxygenation if the risk of bradycardia and desaturation during tracheal intubation are possible. Other adverse effects (bradycardia, propofol infusion syndrome, respiratory depression, immune function) are poorly documented in neonates and require further investigation. Neonates can experience pain on injection and the use of lignocaine to ameliorate this effect is suggested.
Thiopental
Mechanism
Thiopental is an analogue of pentobarbitone. The greater lipid solubility of thiopental is achieved by substituting a sulfur atom in place of an oxygen atom on the barbiturate acid ring [132]. Greater penetration of the BBB has been described in neonates compared with older animals, possibly attributable to the greater blood-brain flow in this cohort [133]. The most likely mechanism of action of thiopental is via binding to GABAA receptors, which increases the duration of GABA-activated chloride opening.
Pharmacodynamics
The ED50 of thiopental varies with age: 3.4 mg/kg in neonates, 6.3 mg/kg in infants, 3.9 mg/kg in children aged 1–4 years, 4.5 mg/kg in children 4–7 years, 4.3 mg/kg in children 7–12 years and 4.1 mg/kg in adolescents aged 12–16 years [134, 135]. It remains to be determined whether altered PK or PD responses explain the reduced dose requirements in neonates. The effect site concentration of thiopental for induction of anesthesia in neonates may be less than that in infants because the neonate has relatively immature cerebral cortical function, rudimentary dendritic arborizations and relatively few synapses [136]. However, integrated PK–PD studies with thiopental in neonates have not been performed to support or refute this premise [137]. The plasma concentration (EC50) of thiopental required for induction of anesthesia in adults based on the EEG is 17.9 mcg/mL; comparable data in neonates are lacking [138]. The T 1/2keo in adults is 0.6 min [138], but there are no estimates in neonates. Children aged 13–68 months given rectal thiopental (44 mg/kg) 45 min prior to surgery were either asleep or adequately sedated with plasma concentrations above 2.8 mcg/mL [139].
Pharmacokinetics
Peak concentrations of thiopental are reached in the brain and other well-perfused organs within one circulation time. Recovery is due to redistribution. Reported pharmacokinetic parameter estimates have been derived from infusions administered for seizure control in neonates suffering hypoxic-ischemic insults. Clearance estimates in neonates ranged from 66 to 320 mL/h/kg with a volume of distribution at steady state (Vss) of 3.6–5.4 L/kg [140–143]. Interindividual variability was considerable [137]. While most clearance estimates are less than those in adults (200 mL/h/kg) [138], interpretation is difficult because the hypoxic-ischemic insult will also affect the clearance. Clearance is through oxidation (CYP2C19) to an inactive metabolite, thiopental carboxylic acid, and neonatal immature hepatic function decreases oxidizing capacity. CYP2C19 microsomal activity is approximately 30 % of mature values in the third trimester and increases dramatically around birth [50]. A recent analysis of thiopental clearance maturation is consistent with the timing of maturation of CYP2C19. Clearance rapidly increases during the neonatal period from 33 mL/h/kg at 24 weeks’ PMA to 160 mL/h/kg at term; an adult clearance of 200 mL/h/kg is reported [144]. Neonates (25.7–41.4 weeks’ PMA) undergoing surgery on the first day of life yielded an elimination half-life of 8 h (interquartile range (IQR), 2.5–10.8) and a clearance of 92 mL/min/kg (IQR 20 100) [145]. Thiopental has a low hepatic extraction ratio (0.3), exhibiting capacity-limited elimination. In adults, 10–12 % of thiopental is metabolized per hour; comparable data are not available in neonates. Michaelis-Menten kinetics are reported after prolonged infusion in adults. Michaelis-Menten kinetics are also reported in neonates. The Michaelis constant (Km 28.3 mg/L) is similar to that reported for adults (26.7 mg/L). The maximum rate of metabolism (Vmax) increases from 0.44 mg/min/kg at 24 weeks’ PMA to 5.26 mg/min/kg at term; an adult Vmax of 7 mg/min/kg is reported [144].
Adverse Effects
These are similar to those described for propofol. Thiopental has little direct effect on vascular smooth muscle tone. Cardiovascular depression is centrally mediated by inhibition of sympathetic nervous activity and direct myocardial depression through effects on trans-sarcolemmal and sarcoplasmic reticulum calcium flux [146]. There is no pain on injection. Because the action of thiopental is terminated by redistribution and metabolism is slow, recovery may be very slow after an infusion of thiopental.
Ketamine
Mechanism of Action
The analgesic properties of ketamine are mediated by multiple mechanisms at central and peripheral sites. The contribution from N-methyl-d-aspartate (NMDA) receptor antagonism and interaction with cholinergic, adrenergic, serotonergic and opioid pathways and local anesthetic effects remain to be fully elucidated.
Pharmacodynamics
Ketamine is available as a mixture of two enantiomers; the S(+)-enantiomer has four times the potency of the R(−)-enantiomer. S(+)-ketamine has approximately twice the potency of the racemate. The metabolite norketamine has a potency that is one-third that of its parent. Plasma concentrations associated with hypnosis and amnesia during surgery are 0.8–4 μg/mL; awakening usually occurs at concentrations less than 0.5 μg/mL. Pain thresholds are increased at 0.1 μg/mL [147]. Data from neonates are not available.
Pharmacokinetics
Ketamine is very lipid soluble with rapid distribution. Ketamine undergoes N-demethylation to norketamine. Elimination of racemic ketamine is complicated by the R(−)-ketamine enantiomer, which inhibits the elimination of the S(+)-ketamine enantiomer [148]. Clearance in children is similar to adult rates (80 L/h/70 kg, i.e., liver blood flow) within the first 6 months of life, when corrected for size using allometric models [35]. Clearance in the neonate is reduced (26 L/h/70 kg) [149–151], while Vss is increased in neonates (3.46 L/kg at birth, 1.18 L/kg at 4 years, 0.75 L/kg at adulthood [135]). This larger Vss in neonates contributes to the observation that neonates require a fourfold greater dose than 6-year-old children to prevent gross motor movement [152]. There is a high hepatic extraction ratio and the relative bioavailability of oral, nasal, and rectal formulations is 30–50 %.
Adverse Effects
Ketamine can cause psychotic reactions and hallucinations that can cause distress in older children. These effects can be attenuated by benzodiazepines. An antisialagogue may be effective to diminish copious secretions that may occur after parenteral administration. Tolerance in children may occur with repeated use. Hypocapnia may attenuate ketamine-induced increases in intracranial pressure.
Ketamine is infrequently used in neonates, with perhaps the exception of those with right-to-left congenital heart defects. However, even this limited use has come under scrutiny over concerns that NMDA antagonists (e.g., ketamine and GABAA agonists) cause significant neuronal apoptosis and other cellular dysgenesis during the period of rapid synaptogenesis in newborn animals [153–155]. Neonatal rats that were anesthetized with ketamine but did not undergo any surgical stimulation or inflammatory response sustained widespread neuronal apoptosis and long-term memory deficits.[156, 157]. The severity of these findings appeared to depend on both the dose and duration of exposure and the coadministration of other proapoptotic compounds in these 7-day-old rats. Moreover, these same findings have been reported in other animals including nonhuman primates and in the presence of anesthetics other than ketamine. More recently, interventions have been forthcoming that appear to prevent or attenuate the neurocognitive sequelae from these anesthetics in newborn animals [158–160]. Nonetheless, extrapolating these animal data to the care of human neonates remains contentious [161, 162].
Inhalation Agents
Although inhalational anesthetics have been administered to children for 150 years, the pharmacology of inhalational anesthetics in neonates has only been the subject of investigation for 50 years or less. Research has clarified the pharmacokinetics of inhalational anesthetics in neonates, their MAC values and cardiorespiratory responses to clarify the pharmacology of inhalational anesthetics in this vulnerable age group. These developments have allowed practitioners to provide safe anesthesia while attenuating the incidence and severity of adverse events.
Physicochemical Properties
The chemical structures of the current inhalational agents are based on a polyhalogenated ether skeleton (with one exception): isoflurane and desflurane are methyl ethyl ether anesthetics and sevoflurane is a methyl isopropyl (Table 3.1). The single exception to the ether skeleton is halothane, a polyhalogenated alkane that is infrequently used today. Desflurane differs from its older cousin, isoflurane, only in the substitution of a fluoride atom for a chloride on the alpha carbon of isoflurane, whereas sevoflurane differs by the substitution of a trifluoromethyl group for chloride on the alpha carbon of isoflurane. The minor atomic substitutions and structural differences among these ether anesthetics confer substantial differences in their physicochemical and pharmacological properties that are elucidated in Table 3.1.
Table 3.1
Pharmacology of inhaled anesthetics
Halothane | Enflurane | Isoflurane | Sevoflurane | Desflurane | |
---|---|---|---|---|---|
Pharmacology | |||||
Chemical structure | ![]() | ![]() | ![]() | ![]() | ![]() |
Molecular weight | 197.4 | 184.5 | 184.5 | 200.1 | 168 |
Boiling point (°C) | 50.2 | 56.5 | 48.5 | 58.6 | 23.5 |
Vapor pressure (mmHg) | 244 | 172 | 240 | 185 | 664 |
Saturation concentration (%) | 34 | 24 | 34 | 26 | 93 |
Odour | Mild, pleasant | Etheric | Etheric | Pleasant | Etheric |
Solubility | |||||
λ b/g adults | 2.4 | 1.9 | 1.4 | 0.66 | 0.42 |
λ b/g neonates | 2.14 | 1.78 | 1.19 | 0.66 | – |
λ brain/b adults | 1.9 | 1.3 | 1.6 | 1.7 | 1.2 |
λ brain/b neonates | 1.5 | 0.9 | 1.3 | – | – |
λ fat/b adults | 51.1 | – | 45 | 48 | 27 |
MAC | |||||
MACadults | 0.75 | 1.7 | 1.2 | 2.05 | 7.0 |
MACneonates | 0.87 | – | 1.60 | 3.2 | 9.2 |
Pharmacokinetics
In the 1960s, investigators determined that the washin curves for halothane (Fig. 3.9) and nitrous oxide in neonates were more rapid than in adults [26, 163]. While the rate of increase of alveolar to inspired partial pressures of nitrous oxide in adults is rapid, achieving an FA/FI ratio of 0.8 within 10 min, it is even more rapid in neonates and infants, achieving a ratio of 0.9 within 5 min. The fundamental principle underlying the pharmacokinetics of these anesthetics in neonates is the movement of inhalational anesthetics among organs in the body. Outside of the body, inhalational anesthetics exist in the gas phase where the concentrations or partial pressures are interchangeable (assuming the ideal gas law). However inside the body, the concentrations of these anesthetics in any liquid or solid tissue exceed the equivalent partial pressure because they are bound to proteins and lipids. In addition, these anesthetics move across membranes (from the functional residual capacity (FRC) to the blood or blood to tissue phases) without impediment, along partial pressure gradients, not concentration gradients. Conceptually, this is identical to our understanding of the movement of other gases such as oxygen and carbon dioxide in the body. Inhalational anesthetics move across membranes seeking to equilibrate partial pressures, even though the concentrations of anesthetics in the tissues differ. As a result, we only refer to inhalational anesthetics in terms of their partial pressures inside the body.


Fig. 3.9
Rate of rise of alveolar to inspired partial pressures of halothane in children and adults (reproduced with permission, Salanitre & Rackow [26])
Four factors explain the more rapid washin of inhalational anesthetics in neonates compared with older children and adults (Table 3.2). The first factor is the delivery of anesthetics to the lungs. Alveolar ventilation determines the rate of delivery of anesthetic to the lungs and thus to the FRC [164]. The greater the ratio of the alveolar ventilation to FRC, the more rapidly the anesthetic partial pressure in the FRC increases. In neonates, this ratio is 5:1, threefold greater than in adults, 1.5:1. The remaining three factors explain the rapid washin of anesthetics in neonates by their effects on the uptake of anesthetics from the lungs. Although a greater cardiac output should actually slow the washin of anesthetic into the FRC, in neonates it speeds the washin. The reason for this is that the greater cardiac output in neonates is primarily distributed to the vessel-rich group (VRG) of tissues (brain, heart, kidneys, and gastrointestinal and endocrine organs), which in the neonate comprises a large fraction (18 %) of the body weight compared with 5 % in the child/adult. Because the VRG receives such a large proportion of the cardiac output, the anesthetic partial pressures in the VRG equilibrate very rapidly, leaving much of the blood returning to the heart with partial pressures that are similar to those in the blood that left the heart. Hence, a diminishing quantity of inhalational anesthetic is taken up from the FRC into the pulmonary blood, allowing the partial pressure in the FRC to increase. At the same time, the blood and tissue solubilities of inhalational anesthetics in neonates are less than those in older children and adults (Fig. 3.10, Table 3.1) [28, 165, 169]. This is true for the more soluble inhalational anesthetics with 18 % less solubilities in neonates compared with older children and adults. However, in the cases of the less soluble anesthetics, sevoflurane and desflurane, the solubilities in neonates likely do not differ substantially from those in adults [28]. Hence, blood solubility differences of the less soluble inhalational anesthetics do not contribute substantively to the rapid washin of sevoflurane and desflurane in neonates. Similarly, age-related differences in hemoglobin, serum concentration of α-1-acid glycoprotein and prematurity do not significantly affect the solubility of most inhalational anesthetics in the blood [166, 167]. Accordingly, the uptake of anesthetics by blood and tissues in neonates is relatively small leaving the partial pressure in the FRC to increase unabated.

Table 3.2
Determinants of the rapid washin of inhalational agents in infants
1. Greater alveolar ventilation to functional residual capacity ratio |
2. Greater fraction of the cardiac output distributed to the vessel-rich group |
3. Reduced tissue/blood solubility |
4. Reduced blood/gas solubility |

Fig. 3.10
Effect of age on the blood/gas partition coefficient of the four inhalational agents, isoflurane, enflurane, halothane and methoxyflurane. The solubility of all four agents in neonates is 18 % less than in adults (reproduced with permission, Lerman et al. [165])
To understand the washin of inhalational anesthetics, it is useful to consider a model such as a reservoir (a sink) to represent the FRC, with water flowing into the sink analogous to alveolar ventilation bringing the anesthetic gases into the FRC. For simplicity, the outlet from the reservoir is plugged in this model. The rate of rise of the level (e.g., washing of anesthetic) follows an exponential curve, the variables of which are determined by the volume of the reservoir and the flow into the reservoir. The equation that describes such a washin is a simple, first-order exponential equation:

where τ is the time constant defined by Eq. (3.2):

Four time constants are required to achieve 98 % equilibration of anesthetic partial pressures in the reservoir. Hence, with an FRC of 0.5 L and an alveolar ventilation of 1 L/min, τ is 0.5 and the time to reach 98 % equilibration of inspired, and alveolar anesthetic partial pressure is 2 min.

(3.1)

(3.2)
Similarly, the increase in anesthetic partial pressure in tissues is determined by a simple first-order exponential curve dependent on the delivery of anesthetic to the tissues (tissue blood flow) and the capacity of the tissues for anesthetic to reach partial pressure equilibration (the product of the volume of the tissue and the solubility of anesthetic in the tissue). This is expressed by an equation similar to that of Eq. (3.2) as follows:

Understanding the washin of inhalational anesthetic into the brain is key to appreciating the pharmacokinetics of induction of anesthesia with an inhalational anesthetic. Assuming the blood flow to the brain is 50 mL/min/100 g of brain (and the brain density is 1 g/mL) and the brain/blood solubility for a particular inhalational anesthetic in an adult is 2.0, then the time constant is
Thus, the time to reach 98 % equilibration of anesthetic partial pressures is 16 min. If the brain/blood solubility were half, 1.0, as might be in the case of a neonate, then the time to 98 % equilibration would decrease by 50 % to 8 min, resulting in a more rapid onset of anesthesia in the neonate and cardiorespiratory sequelae.

(3.3)

The washin curves for the commonly used inhalational anesthetics have been reported for adults [168]. The alveolar to inspired concentrations for halothane reach 0.35 in the first minute of the start of anesthesia, independent of how aggressive the alveolar ventilation (see Fig. 3.11). In the neonate, the washin of halothane in the first minute is probably closer to 0.5, based on the more rapid washin of halothane in neonates [26]. With a maximum inspired concentration for halothane of 5 % and a MAC in neonates of 0.87 %, the alveolar partial pressure would be 5 × 0.5/0.87 or 2.9 × MAC. If sevoflurane were substituted for halothane, then the washin in the first minute would be ~0.5 for both adults and neonates (as sevoflurane is an insoluble anesthetic). With an inspired concentration of 8 % and a MAC of 3.3 %, the alveolar partial pressure would be 8 × 0.5/3.3 or 1.2 × MAC, which is less than one-half that with halothane. Thus, sevoflurane is less likely to cause hemodynamic depression in the neonate in the early period of anesthesia as would halothane, but obversely, sevoflurane does not achieve as deep a level of anesthesia as halothane in the first minute.


Fig. 3.11
Washin of N2O, desflurane, sevoflurane, isoflurane and halothane in adults. The order of washin parallels the solubility of these agents in blood (reproduced with permission from Yasuda N, et al. [168])
The rate of increase of alveolar to inspired partial pressures of inhalational anesthetics varies inversely with the solubility in blood as follows: nitrous oxide > desflurane > sevoflurane > isoflurane > enflurane > halothane > methoxyflurane (Fig. 3.11) [168]. After a stepwise change in the inspired partial pressure of less soluble anesthetics, the alveolar partial pressure equilibrates very rapidly with the new inspired partial pressure. Since the washout of these anesthetics is equally rapid (see below), the inspired partial pressure can be returned to its initial value rapidly by decreasing the inspired partial pressure. Thus, anesthetic depth can be controlled more rapidly with the less soluble than with the more soluble inhalational anesthetics.
The solubilities of the inhalational anesthetics in the vessel-rich tissues in neonates are approximately one-half that in adults (Fig. 3.12) [169]. These reduced tissue solubilities for halothane, isoflurane, enflurane, and methoxyflurane are attributable to two differences in the composition of tissues in neonates compared with those in adults: (1) greater water content and (2) decreased protein and lipid concentrations. The reduced tissue solubilities decrease the time for partial pressure equilibration of anesthetics in tissues (see time constant for tissues, above). Although the partial pressures of inhalational agents in tissues cannot easily be measured in vivo, they may be estimated by measuring the anesthetic partial pressure in the exhaled or alveolar gases. Thus, the reduced tissue solubilities of inhalational anesthetics speed the washin of anesthetic partial pressures in neonates compared with adults.


Fig. 3.12
Effect of age on the solubility of isoflurane, enflurane, halothane and methoxyflurane in the human brain. The solubilities of all anesthetics in the neonatal brain are less than those in older adults (reproduced with permission, Lerman J, et al. [169])
The pharmacokinetics of inhalational anesthetics during the first 15–20 min depends primarily on the characteristics of the vessel-rich group, whereas the pharmacokinetics during the subsequent 20–200 min depends on the characteristics of the muscle group [164]. The solubility of inhalational anesthetics in the skeletal muscle varies directly with age in a logarithmic relationship [169]. This effect of age on the solubility of anesthetics in muscle has been attributed to age-dependent increases in protein concentration in the first five decades of life and in fat content in the subsequent three decades of life [169]. Since the muscle mass in the neonate is small, this effect is attenuated.
The net effect of these differences between neonates and adults is to speed the equilibration of anesthetic partial pressures in alveoli and tissues and thereby speed the rate of equilibration of alveolar to inspired anesthetic partial pressures of soluble anesthetics in neonates compared with adults [26, 163]. However, the difference in the rate of washin of less soluble anesthetics between neonates and adults may be less pronounced than that of more soluble anesthetics.
Ventilation
Changes in alveolar ventilation directly affect the washin of inhalational anesthetics: as alveolar ventilation increases, the washin of the anesthetics increases (Fig. 3.13) [164]. Ventilation is the primary determinant of the delivery of anesthetics to the lungs to effect the washin of the anesthetics. The effect of ventilation on the washin is more pronounced with more soluble anesthetics, e.g., halothane, and less pronounced or limited with less soluble anesthetics, sevoflurane and desflurane. The reason for the differential effect of ventilation rests with the dependency of the anesthetic on its speed of delivery to achieve partial pressure equilibration. Those anesthetics that are more soluble in blood (and tissues) are taken up more rapidly by blood (and tissues), thus slowing their washin. Conversely, those that are less soluble in blood are taken up very little by blood, facilitating a more rapid washin.


Fig. 3.13
Effect of alveolar ventilation on the washin of soluble (ether), intermediate (halothane), and insoluble (N2O) anesthetics. Changes in ventilation affect the washin of more soluble than less soluble anesthetics (reproduced with permission, Eger EI 2nd. Anesthetic uptake and action. Baltimore: Williams & Wilkins; 1974) [164]
Cardiac Output
Changes in cardiac output inversely affect the washin of inhalational anesthetics compared with ventilation: the greater the cardiac output through the lungs, the more rapidly anesthetic is removed from the lungs and the slower the washin of anesthetic into the alveoli [164]. In the neonate, the effect of cardiac output is paradoxical as the greater cardiac output actually speeds the washin since most of the cardiac output is directed to the VRG, which comprises 18 % of the cardiac output, that speeds the equilibration of alveolar to inspired anesthetic partial pressure.
Induction
The more rapid washin of insoluble versus soluble anesthetics is generally believed to parallel a more rapid induction of anesthesia with the former, although this notion is probably untrue. Whereas the washin is determined by the pharmacokinetics of the agents, the speed of induction of anesthesia depends upon both pharmacokinetic and pharmacodynamic factors including: (1) the rate of equilibration of anesthetic partial pressures (determined by the four factors in Table 3.2), (2) the maximum inspired concentration, (3) airway irritability and (4) the MAC value. It is the interaction of these four factors that determines the relative speed of induction of anesthesia.
The rate of washin of inhalational anesthetic into the lungs varies inversely with the solubility of inhalational anesthetics in the blood (N2O > desflurane > sevoflurane > isoflurane > halothane > methoxyflurane) [168]. Only two of these anesthetics are truly devoid of airway irritability when delivered by mask, sevoflurane and halothane. Although less soluble anesthetics washin to the FRC more rapidly than more soluble anesthetics, this more rapid washin is offset by the maximum inspired concentration (overpressure technique) and their greater MAC (Table 3.1). Using Fig. 3.11, we can explain why neonates may appear to be unconscious early in the induction but still respond to painful stimuli as we frequently experience. See below for full discussion.
Control of Anesthetic Depth
Two feedback mechanisms modulate the depth of anesthesia during inhalational anesthesia: respiratory and cardiovascular. During spontaneous ventilation, respiratory depression limits the depth of anesthesia. As the depth of anesthesia increases, alveolar ventilation decreases, the neonate arouses from anesthesia as the anesthetics are redistributed away from the VRG, and spontaneous ventilation increases. This is a negative feedback effect [170]. This protective mechanism permits the use of inspired concentrations of inhalational anesthetics severalfold greater than MAC (overpressure technique) while protecting against excessive circulatory depression. However, if ventilation is controlled, this protective mechanism is bypassed. The alveolar to inspired anesthetic partial pressure ratio increases relentlessly as cardiac output decreases. This decrease in cardiac output limits removal of anesthetic from the lung leading to a further increase in the alveolar partial pressure of anesthetic. This is a positive feedback effect [170]. In such a circumstance, this leads to a downward spiral that might profoundly depress the cardiovascular system and lead to death if the cycle is not interrupted. In dogs breathing spontaneously, concentrations of halothane up to 6 % are tolerated without cardiovascular collapse because the negative feedback mechanism of respiratory depression prevents excessive concentrations within the lungs, whereas if ventilation were controlled, concentrations of halothane ≥4 % will profoundly depress the cardiovascular system and lead to death [170]. Large concentrations of inhalational anesthetics (overpressure technique) are commonly administered during inhalational inductions either as stepwise increases in concentration or as a single-breath large concentration. These large concentrations can be tolerated providing spontaneous ventilation is maintained. If, however, the pattern of ventilation changes from spontaneous to controlled, then circulatory collapse may occur.
This holds particular relevance for neonates. When halothane was administered to neonates, hypotension (and bradycardia) occurred; however, when sevoflurane was introduced, a similar response was not observed, despite the more rapid washin of this new insoluble anesthetic. The reason rests more with the deliverable MAC values for each agent rather than the pharmacokinetics. With a maximum deliverable inspired concentration of 5 % halothane and a MAC in neonates of 0.87 %, 5.7 inspired MAC multiples could be delivered. However, with a maximum deliverable inspired concentration of 8 % sevoflurane and a MAC in neonates of 3.3 %, only 2.4 inspired MAC multiples, less than half that deliverable by halothane, can be delivered to the neonate. These calculations demonstrate the greater safety associated with the use of sevoflurane in neonates compared with halothane.
Shunts
Shunts exist in two forms: left to right or right to left. Left-to-right shunts refer to conditions in which blood recirculates through the lungs (usually an intracardiac defect such as a ventricular septal defect). Right-to-left shunts refer to conditions in which venous blood returning to the heart bypasses the lungs (as in an intracardiac (cyanotic heart defect) or intrapulmonary (pneumonia or an endobronchial intubation) defect). The effects of shunts on the rate of equilibration of alveolar to inspired anesthetic partial pressures are poorly understood. In general, left-to-right shunts do not significantly affect the pharmacokinetics of potent inhalational agents, provided cardiac output remains unchanged. In contrast, right-to-left shunts can significantly delay the washin of inhalational anesthetics [171]. The magnitude of the delay with a right-to-left shunt depends on the solubility of the anesthetic: the less soluble the anesthetic (nitrous oxide, desflurane and sevoflurane), the more delayed the washin compared with that of the more soluble anesthetics. These effects are independent of the anatomical level of right-to-left shunts: intracardiac or intrapulmonary (as in the case of an endobronchial intubation).
To understand why right-to-left shunts affect the pharmacokinetics of inhalational anesthetics and less soluble anesthetics in particular, it is useful to consider a simplified model of the lung in which each lung is represented by one alveolus and each lung is perfused by one pulmonary artery (Fig. 3.14) [27]. When the tracheal tube is positioned with its tip at the mid-trachea level (Fig. 3.14a), ventilation is divided equally between both lungs, thereby yielding equal anesthetic partial pressures in both pulmonary veins (PV = 1). However, if the tip of the tube is advanced into the right bronchus (Fig. 3.14b), all of the ventilation is delivered to one lung, that is, the ventilation to that lung is doubled, whereas ventilation to the non-ventilated lung is zero. Under these conditions, the partial pressure of CO2 remains unchanged. When a soluble anesthetic is administered as in the case of Fig. 3.14b, the partial pressure of anesthetic in the combined pulmonary veins is approximately the same as in the presence of a tracheal intubation. The similar anesthetic partial pressure occurs because the increased ventilation to the ventilated lung compensates to a large extent for the shunt and speeds the increase in alveolar to inspired anesthetic partial pressures. However, when a less soluble anesthetic is administered in the presence of a right-to-left shunt, the effects on the washin of the inhalational agent are quite different. In this case, the increase in ventilation to the ventilated lung minimally increases the washin of the anesthetic (Fig. 3.14c) because changes in ventilation do not substantively affect the washin of insoluble anesthetics (Fig. 3.13). As a consequence, minimal increase in washin to the ventilated lung cannot offset the effects of the shunt. The anesthetic partial pressure in the combined pulmonary vein thus lags behind the partial pressure in the veins when both lungs are ventilated. Induction of anesthesia with a less soluble anesthetic is thereby slower in the presence of a right-to-left shunt than in the absence of a shunt. The less soluble anesthetics, desflurane and sevoflurane, are two such anesthetics whose washin characteristics may be significantly compromised in the presence of a right-to-left shunt.


Fig. 3.14
Effect of shunt on the washin of anesthetic partial pressure in blood. (a) illustrates the normal washin with no shunt, equal ventilation to both lungs and normocapnia. (b) illustrates the effect of a right-to-left shunt (via an endobronchial intubation) on the washin of a soluble anesthetic. Normocapnia is maintained and hypoxic pulmonary vasoconstriction is negligible. The doubled ventilation to the intubated lung offsets the effect of the shunt. The washin is similar to (a). However, (c) illustrates the dramatic effect of a right-to-left shunt on the washin with a less soluble anesthetic. Since the increased alveolar ventilation to the intubated lung does not substantively affect the washin to the lung, when the blood from the ventilated lung combines with that from the shunted lung, the net effect is a slower washin of anesthetic (with permission, Lerman J. [27])
The washin of halothane was recently evaluated in children with fenestrated Fontan before and after closure of the fenestration [171]. The authors noted that before the fenestration was closed (right-to-left shunt open), the washin of halothane as determined by the increase in arterial to inspired partial pressures was delayed compared with that after the fenestration was closed. Closure of the fenestration increased the pulmonary to systemic blood flow ratio from 0.58 to 0.88. Importantly, they noted that the increase in the end-tidal partial pressure of halothane did not parallel the arterial partial pressure. This observation is consistent with published evidence that the correlation between the end-tidal and arterial partial pressures of a gas (carbon dioxide) in children with cyanotic heart disease is poor [172].
Metabolism
The metabolism of inhalational anesthetics is discussed below under Renal and Hepatic Effects.
Emergence
The washout of inhalational agents follows an exponential decay (the inverse of the washin curve) [168]. The order of the washout of the anesthetics parallels their blood/gas solubilities, that is, the anesthetic that is washed out first, desflurane, is the least soluble in blood [168]. The order of washout in children is expected to be similar to that in adults.
Recovery of motor function in rats parallels the washout of inhalational anesthetics from fastest to slowest: desflurane < sevoflurane < isoflurane < halothane [173]. Notably, the rate of recovery increases in parallel with the duration of anesthesia [173]. In studies in which the recovery from two or more inhalational agents was compared, the end-tidal concentrations of the anesthetics were maintained at approximately 1 MAC until the conclusion of surgery, after which the anesthetics were abruptly discontinued [174–176]. In this paradigm, it is not surprising to find that the rates of recovery paralleled the rates of washout, which in turn paralleled the solubilities of the inhalational agents. In clinical practice however, anesthetic concentrations are gradually tapered as the end of surgery approached. This practice may attenuate the differences in the rates of recovery among inhalational agents reported previously.
In neonates, recovery after inhalational anesthesia is somewhat slower than that predicted solely by the washout curves. In part, this area of investigation has been hampered by a lack of consensus on the essential factors associated with emergence in the neonate [177]. Many anesthesiologists have struggled to explain why neonates recover at a slower rate than expected after a brief anesthetic with only inhalational anesthesia. No clear answers to explain this curiosity have been forthcoming.
Pharmacodynamics
In 1969, the MAC of halothane was noted to increase as age decreased [178]. The MAC of halothane reached its maximum value (1.1 %) in the youngest age group, which included 2 neonates and 4 infants 1–6 months of age. Based on this MAC measurement, one study cautioned against the use of halothane in neonates because it caused more hypotension than it did in older infants [179]. We postulated at the time that neonates were more sensitive to the myocardial depressant effects of halothane than children. Subsequently, when we determined the MAC of halothane in neonates as a group distinct from older infants 1–6 months of age, the MAC in the former group, 0.87 SD 0.1 %, was 15–25 % less than that in the latter, 1.2 % [94]. Furthermore, when 1 MAC of inhalational anesthetics was administered, the systolic blood pressure and heart rate responses in neonates were similar to those in older infants [94, 180, 181]. The MAC values in full-term neonates for the inhalational anesthetics in use today are 1.60 SD 0.01 % for isoflurane [182], 9.0 SD % for desflurane [180] and 3.3 SD 0.2 % for sevoflurane [181]. In addition, there is evidence that the primary cause of the increased sensitivity of neonates to cardiodepression by inhalational anesthetics may also be explained by immaturity of the cardiovascular system [183]. Using echocardiography, the cardiodepressant effects of halothane and isoflurane in concentrations up to 1.5 MAC in neonates were noted to be greater than in older infants [184]. The increased susceptibility of neonates to the depressant effects of inhalational anesthetics has been attributed, in part, to a decrease in myocardial contractile elements, a decrease in calcium sensitivity of myocardial fibers [183] and an incomplete sympathetic innervation of the heart and vascular system. Evidence also indicates that both pressor and depressor baroresponses are depressed in the presence of isoflurane in the preterm and full-term neonate [185]. These data suggest that the magnitude of the cardiovascular depression by inhalational anesthetics at 1 MAC in neonates is similar to that reported in older infants and that at concentrations in excess of 1 MAC, it may be greater than in older infants.
In order to attenuate the cardiodepressant effects of inhalational anesthetics in neonates, heart rate should be maintained and preload optimized. Neonatal myocardium evolves during the first month after birth, improving in compliance [186]. Because neonates depend on a rapid heart rate to maintain cardiac output, cardiovascular depression, particularly in the presence of halothane, can be reversed or offset in part by intravenous atropine (0.02 mg/kg) [184, 187]. To optimize preload, balanced salt solution or albumen, in a volume of 5–20 mL/kg, should be administered before anesthesia is induced [93, 184]. Neonates who have had cardiorespiratory dysfunction in the presurgical period or were managed in the neonatal intensive care unit often present to the operating room relatively dehydrated because of aggressive diuretic therapy and/or third-space fluid losses. After rehydration but in the absence of chronotropic agents, systolic arterial pressure decreases ≈ 20–25 % at 1 MAC halothane, desflurane, and sevoflurane compared with awake values in neonates, with either no changes or minimal decreases in heart rate [94, 180, 181]. Similar responses have been reported with 1 MAC of these anesthetics in infants 1–6 months of age.
Curiously, the MAC values for sevoflurane in neonates and infants 1–6 months of age are similar 3.3 and 3.2 %, respectively [181]. It remains unclear why the relationship between the MAC of sevoflurane and age in childhood differs from that for the other inhalational anesthetics. Whether the conformational structure of sevoflurane, a methyl isopropyl ether, or some other physicochemical characteristic is responsible for this unique relationship between MAC of sevoflurane and age is unclear.
The MAC of isoflurane decreases steadily in neonates as gestational age decreases to 24 weeks (Fig. 3.8) [93]. Not only did this study characterize the relationship between the MAC for isoflurane in preterm neonates and age, but it also confirmed the notion that neonates as young as 24 weeks’ gestation respond to noxious stimuli in a predictable manner. Although several explanations have been posited to explain the age-dependent change in MAC in the perinatal period including residual effects of placentally transmitted female hormones, central nervous system substance P and maturation of central nervous system, the cause remains speculative.
Several factors moderate the MAC of inhalational anesthetics in infants and children. The MAC of halothane in children with cerebral palsy and severe mental retardation is approximately 25 % less than that in children without disabilities [188].
Whether these same relationships hold true in neonates has not been established. Acute administration of barbiturates and benzodiazepines decreases MAC [189, 190]; chronic administration of similar medications (such as in the case of children who have been treated with anticonvulsants) does not appear to affect MAC [191]. The effects of specific anticonvulsants such as valproic acid and phenytoin on the MAC of inhalational agents require clarification. Adults who are homozygote for melano-cortin for desflurane require ~20 % more anesthetic (6.2 % vs 5.2 %) than for brunettes [192].
The additivity of MAC fractions of inhalational agents (as well as nitrous oxide) is well established. However, the concept of additivity in children does not hold true for all inhalational agents: the additive contribution for nitrous oxide holds true for the MAC of halothane and isoflurane [193, 194] but not for the MAC of sevoflurane and desflurane [180, 181, 195] children 1–3 years, only 24 %, and that of desflurane only 22 %. Whether the same holds true in neonates is unknown. Additional evidence from the MAC response to tracheal intubation supports this attenuated effect of nitrous oxide on the MAC of sevoflurane in children [196]. This differential effect of nitrous oxide on the MAC values of inhalational agents in children and of its effect on the MAC of sevoflurane and desflurane between children and adults have not been explained.
Nitrous oxide is commonly used to supplement general anesthesia. However, nitrous oxide is infrequently used in neonates, particularly preterm neonates, who require emergency surgery and who are at risk of pulmonary or retinal complications from high oxygen tensions. The avoidance of nitrous oxide in neonates who have bowel obstruction or gas-filled closed spaces is well accepted [197]. However, its avoidance in neonates who are at risk of oxygen toxicity is less clear. It has been recommended that the PaO2 be limited to a maximum value of 80 mm Hg (that is, an SaO2 of 93 %) to prevent the effects of oxygen toxicity. This value lies at the shoulder of the steep descending portion of the oxyhemoglobin dissociation curve. If nitrous oxide were used to maintain the arterial oxygen tension below this value, then any minor difficulty with the airway could lead to a rapid hemoglobin oxygen desaturation. This potential problem would be mitigated if nitrogen were used instead of nitrous oxide, since the former is 34 times less soluble in blood than the latter. In these circumstances nitrous oxide should be avoided and replaced with an air/oxygen mixture.
The MAC responses to stimuli other than skin incision, i.e., tracheal intubation, LMA insertion, extubation and return of wakefulness (MAC awake), have also been determined in children, although their applicability to neonates has not been established [198]. The MAC response to tracheal intubation is 10–50 % greater than the MAC for skin incision for halothane [199, 200], enflurane [201], and sevoflurane [196, 202, 203], whereas the MAC for tracheal extubation is approximately 10–25 % less than the MAC for isoflurane [204], desflurane [205 and sevoflurane [206, 207]. The MAC response to tracheal intubation during sevoflurane anesthesia in children is attenuated in the presence of adjuvants such as clonidine [203]. None of these effects have been validated in neonates.
Central Nervous System
For the past decade, the anesthesia literature has been dominated and preoccupied with the effects of anesthesia on neuroapoptosis in the neonatal brain. Neuroapoptosis is discussed further in Chaps. 4 (Anesthetic techniques for Neonatal Anesthesia) and 15 (Anesthetic Complications in the Neonate) and will not be repeated here.
Although cerebral blood flow (CBF) is autoregulated, there are limits in mean blood pressures beyond which CBF is pressure passive. Evidence suggests that neurological sequelae are increasingly likely when the CBF decreases below 20mL/kg/min. However, there is a dearth of evidence to support this notion in neonates and during general anesthesia [208].
CBF to the cortex is 3–4-fold greater than to white matter, in part, explaining the increasing vulnerability to periventricular leukomalacia in the perinatal period.
All potent inhalational agents depress the central nervous system with dose-dependent decreases in the cerebral vascular resistance and the cerebral metabolic rate for oxygen. The decrease in vascular resistance causes a reciprocal increase in CBF that starts at 0.6 MAC [209]. The extent of the increase in CBF, however, depends on the inhalational agent: halothane > enflurane > isoflurane [209]. The effects of sevoflurane on CBF are similar to those of isoflurane; however, the effects of desflurane differ substantively. Desflurane blunts the cerebral autoregulatory response [210, 211]. The net effect of inhalational agents is an increase in the ratio of the cerebral blood flow to metabolic rate, with desflurane increasing the ratio the greatest.
The effects of inhalational agents on the central nervous system in neonates and children have not been fully elucidated. Preliminary data suggest that cerebral blood flow velocity in children varies directly with the end-tidal carbon dioxide partial pressure during halothane anesthesia [212]. Cerebral blood flow velocity increases as the concentration of halothane increases [213], but does not change significantly in the presence of increasing concentrations of isoflurane.
The EEG activity of desflurane and sevoflurane is similar to that of isoflurane [214, 215]. The electroencephalographic (EEG) activity during halothane anesthesia differs substantially from that during sevoflurane anesthesia [216]. In the case of halothane, the EEG is characterized by slow waves superimposed on fast rhythms (α and β waves), whereas in the case of sevoflurane, the EEG is characterized by mainly sharp slow waves. Furthermore, the shift of power of the EEG from low (1–4 Hz) to medium frequencies (8–30 Hz) is greater for halothane than it is for sevoflurane. The unique EEG tracing in the neonate evolves rapidly in the first few months after birth [217]. During the neonatal period, up to 2 % sevoflurane exerts limited effects on the EEG; however, by 3–5 months of postnatal age, the EEG is responsive to increasing sevoflurane concentrations. The clinical relevance of these EEG differences remains unclear at this time, although the BIS responses, which are derived from the EEG, to sevoflurane differ substantively from those of halothane [105, 218]. Since brain monitors are not used in neonates, this issue is moot.
In children who are anesthetized with sevoflurane, both epileptiform activity in the form of myoclonic movement of the extremities and transient spike and wave complexes on EEG have been reported [219–222].
In two children with histories of epilepsy, diffuse spike and wave complexes were noted on EEG at 5 and 7 % inspired concentrations [220]. In a third child without a history of seizures, a 30-s burst of spike and wave complexes was recorded during sevoflurane anesthesia for spinal surgery but recognized only after the event resolved [222]. None of these three children exhibited clinical evidence of seizure activity. To determine whether these involuntary movements could have as their origin a cortical focus, the EEG was analyzed for evidence of seizure activity in children who had been anesthetized with either halothane or sevoflurane [216]. None of the children displayed either clinical or EEG evidence of seizure activity. The EEG patterns for both halothane and sevoflurane were characteristic even though all of the children had been premedicated with midazolam. The association between myoclonic movement and seizures during sevoflurane anesthesia remains tenuous [223]. A single case of a seizure has been reported in a neonate after sevoflurane anesthesia [224]. However, 3–5 % of children experience at least 1 seizure in childhood, with the greatest incidence occurring in infants <1 year of age [225, 226]. Since epileptiform activity does not portend frank seizures, its clinical relevance during sevoflurane is unknown. Recognizing that idiopathic seizures occur far more frequently than seizures resulting from sevoflurane, the source for such seizures should be sought beyond sevoflurane.
Cardiovascular System
Inhalational agents affect the cardiovascular system either directly (by depressing myocardial contractility or the conduction system or by dilating the peripheral vasculature) or indirectly (by affecting the balance of parasympathetic and sympathetic nervous systems and neurohumoral, renal or reflex responses). The cardiovascular responses to inhalational agents in children are further complicated by maturational changes in the cardiovascular system and its responsiveness to these anesthetics [183, 186]. This is particularly a concern in neonates, although few studies have specifically addressed myocardial contractility in the neonate.
Assessment of cardiovascular variables in infants and children presents a challenge for clinicians. Blood pressure may be measured either invasively (arterial line) or noninvasively. Electrocardiography is routinely used in all age groups to detect arrhythmias. In contrast to blood pressure and electrocardiography, measurement of cardiac output and myocardial contractility is much more difficult to quantify in this age group. Two-dimensional echocardiography and impedance cardiometry have been used to estimate cardiac output and myocardial contractility in infants and children [184, 227–229], although the echocardiographic measurements are subject to variability depending on the preload and afterload. Load-independent derived echocardiographic variables (stress-velocity and stress-shortening indices) have since improved the accuracy of echocardiographic estimates of myocardial function and are used with increasing frequency [230].
In neonates, several factors affect the blood pressure responses to inhalational agents including the particular inhalational anesthetic, the dose, the preload status of the infant, the presence of coexisting diseases and the techniques used to measure the systemic pressure (invasive vs noninvasive) and cardiac function (echocardiography). Most studies demonstrated modest, dose-dependent decreases in blood pressure with all of the inhalational agents. At ~1 MAC, systolic blood pressure decreased ~24 % with halothane, 30 % with sevoflurane and 34 % with desflurane [94, 180, 181]. Systolic pressures may further decrease with concentrations up to 1.5 MAC. Few data exist regarding the hemodynamic responses beyond 1.5 MAC. On balance, all of the inhalational agents (in concentrations up to 1.5 MAC) modestly depress the systemic blood pressure with increasing dose.
The effects of inhalational anesthetics on myocardial contractility and cardiac output in the neonate are incompletely understood. Cardiac output in neonates decreases at 1.0 and 1.5 MAC halothane and isoflurane similarly [184]. Knowing the limited effects of sevoflurane and desflurane on myocardial contractility, one might expect that these anesthetics decrease cardiac output and myocardial contractility at 1 and 1.5 MAC less than halothane [231]. The effects of large concentrations of sevoflurane and desflurane on cardiac function in neonates have been difficult given very large MAC values in this age group. Intravenous atropine and a bolus of balanced salt solution restore, in part, the depressed circulation in neonates [187, 232, 233].
The mechanism by which inhalational agents depress myocardial function remains controversial. Studies in both animal and human myocardial cells suggest that the potent inhalational agents, halothane, isoflurane and sevoflurane, directly depress myocardial contractility by decreasing intracellular Ca2+ flux. Inhalational agents decrease the Ca2+ flux by their action on the calcium channels themselves, the ion exchange pumps and the sarcoplasmic reticulum [183]. Inhalational agents may also attenuate contractility of ventricular myocytes via voltage-dependent L-type calcium channels (which are responsible for release of large amounts of calcium from the sarcoplasmic reticulum) [183, 186, 234, 235].
That neonates and infants are more sensitive to the depressant actions of inhalational agents than older children is supported by experimental evidence of maturational differences between neonatal and adult rat, rabbit, and feline myocardium [235–238]. Structural differences that may account, in part, for the changes in myocardial sensitivity to inhalational agents with age include a reduction in contractile elements, immature sarcoplasmic reticulum and functional differences in calcium sensitivity of the contractile elements, calcium channels and the sodium–calcium pump in the neonatal myocardium [183, 234–236, 238–241]. The determinants of Ca2+ homeostasis in ventricular myocardial cells in the neonate depend on trans-sarcolemma Ca2+ flux to a far greater extent than on the sarcoplasmic reticulum [183]. This is based upon a growing body of experimental evidence that includes the finding that the concentration of the Na+–Ca2+ exchange protein in the neonatal myocardium, a protein that regulates trans-sarcolemma flux of Ca2+, exceeds that in adult cells by 2.5-fold and that its concentration decreases with age as the concentration of voltage-dependent L-type calcium channel increases [236]. Furthermore, halothane reversibly inhibits the Na+–Ca2+ exchange protein in immature myocardial cells [236]. The sarcoplasmic reticulum is poorly developed in neonatal myocardial cells, and this finding weighs heavily against the SR being the major source of Ca2+ required for myocardial contractility.
The baroreflex response is also depressed in neonates with both halothane [242] and isoflurane [185], albeit to a greater extent with the former than the latter. In view of the greater incidence of hypotension in neonates and infants than older children, an intact baroreflex could offset, in part, the cardiovascular consequences. However, inhalational agents blunt this response, leaving the infant vulnerable to the cardiovascular depressant actions of inhalational agents. Prophylactic anticholinergics and preload augmentation may attenuate the decrease in cardiac output in the presence of inhaled anesthetics.
Inhalational anesthetics vary in their effect on cardiac rhythm. Halothane may slow the heart rate, in some cases leading to junctional rhythms, bradycardia and asystole. These are dose-dependent responses. Three mechanisms have been proposed to explain the genesis of halothane-associated dysrhythmias: a direct effect on the sinoatrial node, a vagal effect, or an imbalance in the parasympathetic/sympathetic tone. It has also been suggested that the etiology of the bradycardia during halothane anesthesia may be a withdrawal of sympathetic tone. Bradycardia is particularly marked in the neonate, presumably because parasympathetic influences predominate over the sparse sympathetic innervation of the myocardium in this age group. Junctional rhythms are also common during halothane anesthesia. Atrial or ventricular ectopic beats are rare except in the presence of hyper- or hypocapnia. In infants anesthetized with halothane, 10 μg/kg atropine increases heart rate ≥50 % and promotes sinus rhythm [243]. This dose of atropine also increases blood pressure in infants ≥6 months of age and children.
Halothane also sensitizes the myocardium to catecholamines, particularly during hypercapnia. It decreases the threshold for ventricular extrasystoles during epinephrine administration by threefold [244–246]. In contrast, isoflurane, desflurane and sevoflurane maintain or increase heart rate during the early induction period of anesthesia [176, 180, 181, 227, 229, 231, 247–250]. When bradycardia occurs in an anesthetized neonate, the primary cause is always hypoxia, even during anesthesia, before other causes such as a direct drug effect are given serious consideration. The ether anesthetics, isoflurane, desflurane and sevoflurane, do not sensitize the myocardium to catecholamines to the same extent as halothane [244, 245, 251]. The mechanism by which the sinus node controls automaticity is incompletely understood but may include K currents, hyperpolarization-activated current and T and L forms of Ca2+ currents [183]. Moreover, developmental changes in these channels likely account, in part, for the differential effects of inhalational agents on heart rate with age [239].
Respiratory System
Inhalational agents significantly affect respiration in infants in a dose-dependent fashion via effects on the respiratory center, chest wall muscles, and reflex responses. Halothane depresses respiration by decreasing tidal volume and attenuating the response to carbon dioxide [252–254]. This depression is offset, in part, by an increase in the respiratory rate [253, 254]. These ventilatory responses to halothane are age dependent; minute ventilation in infants decreases to a greater extent than in children [255]. In infants and young children, intercostal muscle activity is inhibited at greater concentrations than the diaphragm [252, 256]. This effect is most pronounced in preterm and full-term neonates and infants and when an endotracheal tube is used in place of an LMA [257]. Isoflurane, enflurane, sevoflurane and desflurane depress the ventilatory drive and tidal volume and attenuate the respiratory responses to carbon dioxide [253, 254, 258–264]. The increase in respiratory frequency that follows respiratory depression may not restore minute ventilation to preanesthetic levels.
Sevoflurane depresses respiration to a similar extent as halothane up to 1.4 MAC, but depresses respiration to a greater extent than halothane at concentrations >1.4 MAC in adults [260]. Sevoflurane does not decrease the tone of the intercostal muscles to the same extent as halothane [256, 260]. The compensatory changes in respiratory rate differ among the anesthetics; respiratory rate increases at ≥1.4 MAC halothane, is unchanged with isoflurane but decreases at ≥1.4 MAC enflurane [253, 254]. When 8 % sevoflurane was compared with 5 % halothane, minute ventilation and tidal volume decreased and respiratory rate increased with both agents to similar extents [265].
Renal
The potent inhalational agents may affect renal function via four possible mechanisms: cardiovascular, autonomic, neuroendocrine and metabolic. Although the first three mechanisms pose no direct threat to renal function, the fourth mechanism, metabolism, is a serious clinical concern that has resulted in renal dysfunction and death after some inhalational anesthetics.
Inhalational agents are metabolized in vivo to varying extents (Table 3.3). Halothane is the inhalational anesthetic still in use that is most metabolized, but releases very little fluoride in the inorganic form. Most of the fluoride that is liberated from the metabolism of halothane exists in an organic form, trifluoroacetate. This compound has been linked to halothane hepatitis (see below). Metabolism of inhalational agents by the CYP450 2E21 releases inorganic fluoride [266]. Sevoflurane releases the most fluoride, followed by isoflurane and desflurane based on the extent of metabolism (Table 3.3). Even after 131 MAC·h isoflurane, the cumulative inorganic fluoride concentration is small [267]. The metabolism of sevoflurane yields both an inorganic and organic fluoride moiety [268]. The organic form, hexafluoroisopropanol (HFIP), is rapidly conjugated and excreted by the kidneys [268]. It poses no serious threat to renal function in humans; however, inorganic fluoride that is released from these three ether anesthetics has garnered great interest in the relationship between inhalational anesthetics and renal function.
Table 3.3
In vivo metabolism of inhalational agents
Inhalational agent | % Metabolized |
---|---|
Methoxyflurane | 50 % |
Halothane | 20 % |
Sevoflurane | 5 % |
Enflurane | 2.4 % |
Isoflurane | 0.2 % |
Desflurane | 0.02 % |
Peak plasma concentrations of inorganic fluoride after exposure to inhalational agents follow an order that is similar to that in Table 3.3: methoxyflurane > sevoflurane > enflurane > isoflurane > halothane ≈ desflurane [269–273]. In the case of methoxyflurane, two metabolites are produced: inorganic fluoride and oxalic acid. Both have been implicated in the pathogenesis of renal dysfunction although clinically, the renal injury was more consistent with the concentration of inorganic fluoride rather than oxalic acid [274, 275]. Subsequent studies demonstrated that >2.5 MAC·h methoxyflurane resulted in subclinical nephrotoxicity provided the plasma concentration of inorganic fluoride exceeded 50 μM and that >5 MAC·h resulted in frank nephrotoxicity if the concentrations exceeded 90 μM [275a]. These clinical concerns led to the voluntary withdrawal of methoxyflurane from clinical practice.
In contrast to the adult experience with methoxyflurane, renal dysfunction was not a feature after this anesthetic in children. The peak plasma concentrations of inorganic fluoride in children anesthetized with methoxyflurane were significantly less than that in adults after an equivalent anesthetic exposure [276]. The reduced plasma concentrations of fluoride in children were attributed to several possible factors including a decreased metabolism of methoxyflurane, a greater uptake of fluoride by bone, an increased excretion of fluoride ions or a reduced renal sensitivity to fluoride in children [276]. Another plausible explanation for the reduced plasma concentrations of inorganic fluoride in children may have been an immature CYP4502E1 isoenzyme system in the kidneys (see below).
That the plasma concentrations of inorganic fluoride in children who were anesthetized with sevoflurane were similar to or greater than those after enflurane raised concerns about possible renal dysfunction after prolonged exposure to sevoflurane [277–279]. Preliminary evidence suggested that inorganic fluoride should not be a serious clinical problem in children since the peak plasma concentrations of inorganic fluoride after sevoflurane anesthesia were similar to those in adults: <20 μM after ≈ 1 MAC•h, which decreased to <10 μM by 4 h after discontinuation of anesthesia [279].
However, peak plasma concentrations of inorganic fluoride paralleled the MAC·h exposure to sevoflurane in both children and adults [279]. Concerns regarding the risk of renal dysfunction after sevoflurane were heightened after reports that the peak plasma concentration of inorganic fluoride in some adults exceeded the purported threshold for nephrotoxicity (90 μM). Despite the large plasma concentrations of inorganic fluoride after sevoflurane anesthesia, there was no evidence of renal dysfunction.
To understand anesthetic-induced nephrotoxicity, it was suggested that the primary isoenzyme responsible for the degradation of enflurane, isoflurane, sevoflurane and methoxyflurane anesthetics was CYP450 2E1 [266, 280–282]; secondary isoenzymes implicated in renal defluorination include CYP450 2A6 and 3A [280]. Subsequently, large quantities of CYP450 2E1 were found not only within the liver (where degradation of ether inhalational agents yielded large plasma concentrations of inorganic fluoride) but also within the kidneys [86]. The affinity of renal CYP450 2E1 for methoxyflurane was found to be fivefold greater than that for sevoflurane [86]. This provided further evidence that the renal dysfunction after ether inhalational agents was likely due to the local production of inorganic fluoride within the renal medulla rather than excessive plasma concentrations of inorganic fluoride. Because CYP450 2E1 has a greater affinity for methoxyflurane than sevoflurane, this explains why renal dysfunction occurred after methoxyflurane and not sevoflurane and was independent of the circulating inorganic fluoride concentrations [86, 283]. Consequently, there is no restriction on the duration of sevoflurane exposure with respect to the risk of fluoride-induced renal dysfunction.
Sevoflurane may also indirectly affect renal function through by-products of its metabolism in the presence of carbon dioxide absorbents, soda lime. These by-products are five in number, but two are potentially nephrotoxic compounds, known as compounds A and B. Compound A is produced in concentrations that may achieve toxicity (at least in rats), whereas B achieves much smaller concentration. Compound A is nephrotoxic in rats, but has not been associated with nephrotoxicity in humans. It is the reason the fresh gas flow has been set to a minimum value of 2 L/min in the presence of sevoflurane in some countries (see below),, although this is a moot point if absorbents devoid of sodium hydroxide and potassium hydroxide (e.g., Amsorb®) are used (see below).
Hepatic
In vivo metabolism of inhalational agents varies with age; increasing within the first two years of life to reach adult values. The developmental changes in metabolism may be attributed to several factors including reduced activity of the hepatic microsomal enzymes, reduced fat stores, and more rapid elimination of inhalational agents in infants and children compared to adults. Halothane, isoflurane, enflurane, sevoflurane and desflurane have all been associated with postoperative liver dysfunction and/or failure [284–288]. Halothane and sevoflurane have also been associated with transient hepatic dysfunction in infants and children, but not in neonates [289–293, 749]. Several case reports of transient postoperative liver failure and one case of fulminant hepatic failure and death have been attributed to “halothane hepatitis” that was confirmed serologically with antibodies to halothane-altered hepatic cell membrane antigens in children [289]. The exact mechanism of the hepatic dysfunction after halothane remains unclear, although some have speculated that it is caused by an immunologic response to a metabolite of halothane. This putative toxic metabolite, a trifluoroacetyl halide compound, is produced during oxidative metabolism of halothane. It is believed that this compound induces an immunologic response in the liver by binding covalently to hepatic microsomal proteins thereby forming an immunologically active hapten. A subsequent exposure to the inhalational agent then incites an immunologic response in the liver [294]. Hepatic enzymes may also be induced by previous administration of drugs such as barbiturates, phenytoin and rifampin. Although some have admonished clinicians of the risks of repeat anesthetics with halothane in children, in view of the millions of uneventful repeat halothane anesthetics in infants and children worldwide, there is insufficient evidence at present to support such an admonition in this population.
Clinical Effects
Induction Techniques
Although the physicochemical characteristics of the ether series of anesthetics would predict that anesthesia could be induced smoothly and more rapidly with these agents than with halothane (Table 3.1) [165, 169], this has not proved to be the case. All of the methyl ethyl ether anesthetics irritate the upper airway in children resulting in a high incidence of breath-holding, coughing, salivation, excitement, laryngospasm and hemoglobin oxygen desaturation [175, 247, 295–300].
Consequently, most clinicians avoid inhalational inductions with these anesthetics.
In contrast to the irritant airway effects of the methyl ethyl ether anesthetics, the methyl isopropyl ether anesthetic, sevoflurane, is well tolerated when administered by mask to infants and children at any concentration [174, 176, 183, 301–311]. The incidences of coughing, breath-holding, laryngospasm, and hemoglobin oxygen desaturation during inhalational inductions with sevoflurane whether by slow incremental increases in concentration or a single breath are similar to those that occur during inductions with halothane. The observation that the airway reflex responses are infrequent after a single-breath induction with 8 % sevoflurane or 5 % halothane casts doubt on the adage that high concentrations of inhalational agents trigger airway reflex responses [306]. In fact, the induction is so smooth with sevoflurane even in neonates that dialling 8 % sevoflurane in one step is routine to achieve rapid induction of anesthesia in the neonate without triggering airway reflexes. By increasing the inspired concentration in one step, the period of excitement or agitation during induction is minimized.
Both intravenous and inhalational agents have been used for induction of anesthesia in neonates with congenital heart disease. Sevoflurane compares favorably with halothane for induction of anesthesia in such patients scheduled for cardiac surgery and may be preferred because it maintains cardiovascular stability to a greater extent than halothane, but similar to isoflurane [312–314].
Central Neuroexcitation
Paroxysmal increases in blood pressure (both systolic and diastolic pressures) and heart rate have been reported in adults after a rapid increase in the inspired concentration of isoflurane or desflurane [315–317]. Neuroexcitatory responses have not been reported in neonates with either of these agents nor have they been reported during sevoflurane or halothane anesthesia at any age [317]. This rapid increase in the inspired concentration of isoflurane or desflurane triggers a massive sympathetic response, mediated by norepinephrine and/or epinephrine, and results in tachycardia and hypertension [318, 319]. Further increases in the inspired concentration of the inciting agent, in an effort to control the tachycardia and hypertension, will not control these responses but will perpetuate or possibly augment the response. In order to restore normal vital signs, the inciting agent must be discontinued and replaced with another inhalational or intravenous agent. Repetitive small increases (1 %) in the inspired concentration of the putative agent produce transient but attenuated catecholamine bursts and cardiovascular responses compared with larger increases in concentration [320, 321]. Fentanyl (2 μg/kg), esmolol and clonidine have all been effective in preventing, attenuating or eliminating these sympathetic responses [322–324]. The site or sites responsible for triggering a neuroexcitatory response are unknown, although the rapidity of the response suggests that the lung is a primary site [325]. Others, however, dispute this notion contending that two sites must be responsible for triggering the sympathetic discharge, the lung and a vessel-rich organ [326]. Of these two sites, the vessel-rich organ, is believed to mediate the greater response [326].
Emergence
Emergence or recovery has been arbitrarily divided into early (extubation, eye opening following commands) and late (drinking, discharge time from recovery or hospital). Although most studies have demonstrated a more rapid early recovery after less soluble anesthetics [230, 301, 327–329], few have demonstrated a more rapid late recovery with these anesthetics than the more soluble anesthetics [174, 176, 330, 331]. Clinicians have been at a loss to explain why neonates/infants with pyloric stenosis recover slowly after a pure inhalational anesthetic. In theory, they should recover rapidly based on the rapid washout of the sevoflurane; however, this has not been our experience. Recovery only appears rapid if desflurane is used without opioids.
The speed of recovery from anesthesia should follow the order of washout of the inhalational agents: desflurane > sevoflurane > isoflurane > halothane > methoxyflurane [168].
Those agents with lower solubility in blood and tissues are eliminated more rapidly than those with greater solubility; the contribution of metabolism to recovery is minor because the relative rate of metabolism is small compared with the duration of exposure to anesthetics. Although some advocate switching inhalational agents from a more soluble to less soluble agent toward the end of surgery for economy and to facilitate a rapid emergence, the only evidence suggests that switching from isoflurane to desflurane 30 min before the end of anesthesia in adults does not speed emergence [332]. However, these results were based on specific set of clinical conditions in adults that may not be applicable to all conditions or to neonates.
The incidence of complications such as airway reflex responses and vomiting during emergence from anesthesia is similar with all inhalational agents [248, 296, 301, 303, 328–331, 333]. In the case of neonates, airway reflex responses are very common, whereas vomiting and emergence delirium after anesthesia are not.
Emergence Delirium
The introduction of the new inhalational agents, desflurane and sevoflurane, has rekindled interest in a clinical entity known as “emergence delirium”. Emergence delirium is defined as a dissociated state of consciousness in which a child is inconsolable, irritable, uncompromising and/or uncooperative. The child is often demanding that all monitors and all bandages be removed and that they be dressed in their own clothing. Parents who witness this transient state, which lasts 10–20 min and occurs primarily in preschool age toddlers, usually volunteer that this behavior is unusual and uncustomary for their child. However, emergence delirium is rare and unreported in neonates. The incidence of emergence delirium after isoflurane, sevoflurane, and desflurane appears to be similar, but significantly greater than after halothanef [301, 328, 329, 331, 334–336]. The etiology of emergence delirium is unknown. Although pain was initially thought to be responsible for triggering this disorder, a report of a high incidence of delirium after sevoflurane anesthesia for MRI established that pain was not the primary causative agent of delirium after these newer anesthetics [337]. The diagnosis of delirium after inhalational anesthesia was bolstered by the introduction of the Pediatric Anesthesia Emergence Delirium (PAED) scale [338], although this scale has not been validated in neonates. Several preventative measures and interventions have been proposed for the child with delirium after anesthesia [339].
Neuromuscular Junction
Inhalational agents potentiate the actions of non-depolarizing muscle relaxants [340–342] and decrease neuromuscular transmission [343], the latter however only at large concentrations. The mechanism of the reduced neuromuscular transmission is unknown but may be arise from the depression of the central nervous system. The mechanism of the potentiation of non-depolarizing muscle relaxants by inhalational agents is also unknown but is likely attributable to actions of inhalational agents at the neuromuscular junction rather than pharmacokinetic or central nervous system effects. The potentiation of action of non-depolarizing relaxants follows the order: isoflurane ≈ desflurane ≈ sevoflurane > enflurane > halothane > nitrous oxide/narcotic technique [340, 344]. However, this potentiation may depend on the type of non-depolarizing relaxant studied (longer-acting relaxants are affected to a greater extent than intermediate-acting relaxants) [340, 341, 345] and the concentration of inhalational agent (smaller concentrations may not demonstrate any differences among inhalational agents, whereas greater concentrations may demonstrate differences) [341]. During 0.6 MAC isoflurane, it took 56 min after 0.45 mg/kg and 100 min after 0.6 mg/kg rocuronium to recover the first twitch to 75 % of baseline [346].
Malignant Hyperthermia
All inhalational anesthetics (except xenon) trigger MH reactions in susceptible patients [347–356]. To date, MH has not been reported in a neonate. Nonetheless, neonates with a family history of MH should be managed with standard MH precautions. For the management of MH-susceptible patients, the reader should refer to the MHAUS website (http://www.MHAUS.org) or the local national MH website.
Stability
Inhalational agents may be degraded via one of several pathways in the presence of most CO2 absorbents to form several potentially toxic by-products. Enflurane, isoflurane and desflurane (but not halothane and sevoflurane) react with desiccated soda lime to produce carbon monoxide. Both halothane and sevoflurane may be degraded when incubated with some CO2 absorbents, yielding compounds that are potentially organ toxic (see Canizarro reaction below) [357]. Two new absorbents may address these clinical risks: molecular sieves [358] and absorbents lacking sodium and potassium accelerants (e.g., Amsorb™) [359–361]. Amsorb™ absorbs CO2 without releasing carbon monoxide or compound A [359, 360]. Carbon dioxide absorbents differ in their composition and, therefore, in their affinity to interact with inhaled agents. Soda lime contains 95 % calcium hydroxide, either sodium or potassium hydroxide, and the balance as water. Baralyme contains 80 % calcium hydroxide, 20 % barium hydroxide and the balance as water. Amsorb™ contains 70 % calcium hydroxide, 0.7 % calcium chloride, 0.7 % calcium sulfate, 0.7 % polyvinylpyrrolidone and the balance as water. Amsorb™ and other nonreactive absorbents contain neither sodium nor potassium hydroxide, compounds added to improve CO2 absorption efficiency [360, 362].
Carbon monoxide may be released into the anesthetic breathing circuit when isoflurane or desflurane is incubated with a desiccated CO2 absorbent. The absorbent within a CO2 canister may become desiccated if dry fresh gas flows through the canister at a rate sufficient to remove most of the moisture (i.e., >5 L/min continuously through the absorbent canister for 24 h or greater while it is not in service). If the circuit reservoir bag is detached from the canister while fresh gas is flowing, then gas flows through the canister and exits through two sites: a smaller fraction of the fresh gas exits through the inspiratory limb of the canister and a larger fraction of gas flows retrograde through the canister and exits at the site where the reservoir bag should be attached. If the reservoir bag is attached to the canister, then less gas flows retrograde through the canister and the time to desiccate the absorbent is markedly prolonged. Once the absorbent becomes desiccated, carbon monoxide may be released into the inspiratory limb of the breathing circuit if one of the methyl ethyl ether inhalational agents (desflurane, isoflurane or enflurane) is administered [363–365]. The magnitude of the carbon monoxide production follows the order from greatest to least: desflurane ≥ enflurane > isoflurane > > halothane = sevoflurane. Other factors that determine the magnitude of the carbon monoxide concentration produced include the concentration of the inhalational agent, the dryness of the absorbent, the type of absorbent, and the temperature of the absorbent [363]. Currently, carbon monoxide is not detectable by most freestanding anesthetic agent analysers, pulse oximetry or blood/gas analysers (with the exception of co-oximeters), although it is detectable by mass spectrometry. The key to this problem is prevention: turn off the anesthetic machine at the end of the day, disconnect the fresh gas hose to the absorbent canister, leave the reservoir bag connected to the canister, and avoid contact between the desiccated absorbent and the three ether anesthetics, desflurane, enflurane and isoflurane. Others have suggested that high-flow anesthesia should be avoided whenever a circle circuit is used to prevent inadvertent desiccation of absorbent. If the absorbent is desiccated, then some recommend “rehydrating” the absorbent, although this too is fraught with potential problems (including clumping of the absorbent) [366]. If in fact, the absorbent is suspected of being desiccated, then the authors recommend replacing the absorbent before introducing an inhalational agent. Alternatives to conventional absorbents such as the molecular sieve and Amsorb™ may very well obviate all reactions that occur with current absorbents [359, 360]. When these same ether anesthetics are incubated with desiccated Amsorb™, carbon monoxide is not produced [359, 360]. The incidence of carbon monoxide poisoning during anesthesia remains an extremely rare complication when soda lime is used as the CO2 absorbent. In contrast, the incidence of carbon monoxide poisoning is zero with Amsorb™.
Sevoflurane is both absorbed and degraded via the Cannizzaro reaction in the presence of absorbent resulting in five degradation products [367, 368]. Although the degradation of sevoflurane by the absorbent was initially posited to delay the washin of sevoflurane, recent evidence suggests that the quantity of sevoflurane degraded is of little clinical significance to the speed of washin of sevoflurane [369]. Of the five degradation products between sevoflurane and soda lime, compounds A and B appear in the greatest concentrations in the inspired limb of the breathing circuit. Compound A, fluoromethyl-2,2-difluoro-1-(trifluoromethyl) vinyl ether (also known as PIFE), is nephrotoxic in rats at concentrations ≥100 ppm and has an LC50 of 1100 ppm. Compound B, a methoxyethyl ether compound that is minimally volatile at room temperature, is present in closed circuits at <5 ppm and poses little risk to animals and humans. The remaining three metabolites, compounds C, D, and E, are present in such low concentrations in the breathing circuit that they do not merit further consideration. In a low-flow closed circuit model with an inspired concentration of 2.5 % sevoflurane, the concentration of compound A peaks at 20–40 ppm after several hours of anesthesia [369–373]. Studies are conflicting regarding the risks of using fresh gas flows <2 lpm with sevoflurane and in patients with pre-existing renal dysfunction [374]. In children, compound A concentrations are ≤16 ppm after 5.6 MAC·h sevoflurane in a closed circuit with 2 L/min fresh gas flow [375]. Factors that are known to increase the production of compound A include an increase in the inspired concentration of sevoflurane, Baralyme > soda lime and an increase in the temperature of the absorbent [367, 368].
In contrast, similar studies in humans have yielded inconsistent results [370–372, 379]. The mechanism behind compound A-induced nephrotoxicity is believed to be β-lyase-dependent metabolism to nephrotoxic fluorinated compounds, although this has been the subject of intense debate [283, 380, 381]. More recent evidence suggests that differences in the evidence of nephrotoxicity from compound A in rats and humans relate to differences in metabolic pathways between species [382, 383].
At the present time, sevoflurane is the only inhalational agent for which some federal authorities have recommended minimum fresh gas flow guidelines when it is administered in an anesthetic circuit with soda lime. The minimum fresh gas flow guideline of 2 L/min for >1 h of anesthesia is mandated in only five countries worldwide. The remaining countries where sevoflurane is used have no restriction on the minimum fresh gas flow that can be used in a closed circuit.
Analgesic Drugs
Acetaminophen (Paracetamol)
Mechanism of Action
Acetaminophen is widely used in the management of pain, but lacks anti-inflammatory effects. Acetaminophen has a central analgesic effect that is mediated through activation of descending serotonergic pathways. Prostaglandin H2 synthetase (PGHS) is the enzyme responsible for metabolism of arachidonic acid to the unstable prostaglandin H2. The two major forms of this enzyme are the constitutive PGHS-1 (COX-1) and the inducible PGHS-2 (COX-2). PGHS comprises two sites: a cyclooxygenase (COX) site and a peroxidise (POX) site. The conversion of arachidonic acid to PGG2, the precursor of the prostaglandins (Fig. 3.15), depends on a tyrosine-385 radical at the COX site. Formation of a ferryl protoporphyrin IX radical cation from the reducing agent Fe3+ at the POX site is essential for the conversion of tyrosine 385 to its radical form. Acetaminophen acts as a reducing cosubstrate on the POX site and reduces the availability of the ferryl protoporphyrin IX radical cation (Fig. 3.16). This effect can be reduced by the presence of hydroperoxide-generating lipoxygenase enzymes within the cell (peroxide tone) or by swamping the POX site with substrate such as PGG2. Peroxide tone and swamping explain acetaminophen’s lack of peripheral analgesic effect, platelet effect and anti-inflammatory effects. Alternatively, acetaminophen effects may be mediated by an active metabolite (p-aminophenol). P-Aminophenol is conjugated with arachidonic acid by fatty acid amide hydrolase to form AM404. AM404 exerts its effect through cannabinoid receptors [384].



Fig. 3.15
Phospholipid metabolism to the prostaglandins

Fig. 3.16
Prostaglandin H2 synthetase (PGHS) is the enzyme responsible for metabolism of arachidonic acid to the unstable prostaglandin H2. Formation of tyrosine-385 radical (Tyr385*) at the COX site is dependent on the reduction of a ferryl protoporphyrin IX radical cation (Fe4+ = OPP*+) at the POX site. Paracetamol is a reducing cosubstrate that partially reduces Fe4+ = OPP*+, decreasing the amount available for regeneration of Tyr385*. Figure adopted. Aronoff DM, Oates JA, Boutaud O. New insights into the mechanism of action of acetaminophen: Its clinical pharmacologic characteristics reflect its inhibition of the two prostaglandin H2 synthetases. Clin Pharmacol Ther 2006;79:9–19
Analgesic Pharmacodynamics
Acetaminophen is believed to be an effective antipyretic at serum concentrations of 10–20 mg/L, and these concentrations have been extrapolated to those that provide analgesia. However, the often-cited source for the antipyretic serum concentrations of 10–20 mg/L makes reference to an unpublished source without further elaboration [385]. The calculated ED50 for rectal acetaminophen to avoid any supplemental opioids after day-stay surgery is 35 mg/kg [386]. Time delays of approximately one hour between peak concentration and peak effect have been reported [387, 750]. Pain fluctuations, pain type and placebo effects complicate interpretation of the clinical studies. Population parameter estimates of a maximum effect of oral acetaminophen are 5.17 (the greatest possible pain relief (VAS 0–10) would equate to an Emax of 10) and an EC50 of 9.98 mg/L [388]. The equilibration half-time (T 1/2keo) of the analgesic effect compartment was 53 min with a target effect compartment concentration of 10 mg/L associated with a pain reduction of 2.6/10 [387, 750]. Recent evidence using IV paracetamol generated somewhat different estimates of population parameters with a maximum effect of 4.15 pain units and an EC50 of 2.07 mg/L. However, the equilibration half-time (T 1/2keo) of the analgesic effect compartment was almost double that for oral acetaminophen, 1.58 h [389].
There are few data examining acetaminophen analgesia in neonates. The existing data suggest poor analgesic effect after oral and rectal formulations, after painful procedures [390, 391], after circumcision [392], and during heel prick [393]. This is in contrast to the documented analgesic effect in infants and children [386, 388, 394]. It is unclear why the analgesic effect of acetaminophen in neonates is so poor, but it may be attributable to inadequate serum concentrations, type of pain stimulus or assessment tools for the discrimination of pain. Intravenous paracetamol has been successfully used for neonatal analgesia in the perioperative period [395, 396].
Pharmacokinetics
The relative bioavailability of rectal to oral acetaminophen formulations (rectal/oral) is approximately 0.5 in children, but the relative bioavailability is greater in neonates and approaches unity [23]. There are two intravenous paracetamol formulations available and care must be taken with choice of formulation [397]. One is an acetaminophen formulation, whereas the other, propacetamol (N-acetylpara-aminophenoldiethyl aminoacetic ester), is a water-soluble prodrug of acetaminophen that can be administered intravenously over 15 min. It is rapidly hydroxylated into acetaminophen (1 g propacetamol = 0.5 g acetaminophen) [67].
Acetaminophen has a pKa of 9.5, and in the alkaline medium of the duodenum, acetaminophen is non-ionized. Consequently, the absorption half-time of the non-ionized form from the duodenum is rapid in children (T 1/2abs 4.5 min) who were given acetaminophen as an elixir [397]. The absorption half-time in infants under the age of 3 months was delayed, (T 1/2abs 16.6 min) consistent with delayed gastric emptying in young infants [20, 21]. Rectal absorption is slow and erratic with large variability. For example, absorption parameters for the triglyceride base were T 1/2abs 1.34 h (CV 90 %) with a lag time before absorption began of 8 min (31 %). The absorption half-life for rectal formulations was prolonged in infants less than 3 months (1.51 times greater) compared with those in older children [66].
Sulfate metabolism is the dominant route of elimination in neonates, while glucuronide conjugation (UGT1A6) is dominant in adults [22]. Glucuronide/sulfate ratios range from 0.12 in preterm neonates of 28–32 weeks’ PCA, 0.28 in those at 32–36 weeks’ PCA [399] and 0.34 in full-term neonates <2 days old [400]. A total body clearance of 0.74 L/h/70 kg at 28 weeks’ PMA and 4.9 (CV 38 %) L/h/70 kg in full-term neonates after enteral acetaminophen has been reported using an allometric ¾ power model [66]. Clearance increases over the first year of life and reaches 80 % of that in older children by 6 months of postnatal age [23, 62]. Similar clearance estimates are reported in neonates after intravenous formulations of acetaminophen with weight accounting for almost 60 % of the variability in clearance with age (Fig. 3.7) [401–403, 751]. The relative bioavailability of the oral formulation is 0.9. The volume of distribution for acetaminophen is 49–70 L/70 kg. The volume of distribution decreases exponentially with a maturation half-life of 11.5 weeks from 109.7 L/70 kg at 28 weeks postconception to 72.9 L/70 kg by 60 weeks [23] reflective of fetal body composition, and water distribution changes over the first few months of life.
Adverse Effects
The toxic metabolite of acetaminophen, N-acetyl-p-benzoquinone imine (NAPQI), is formed by the cytochrome P450s CYP2E1, 1A2, and 3A4. This metabolite binds to intracellular hepatic macromolecules to produce cell necrosis and damage. Infants less than 90 days’ PNA have decreased expression of CYP2E1 activity in vitro compared with older infants, children, and adults [53, 404]. CYP3A4 appears during the first week after birth [404], whereas CYP1A2 appears later [54, 404]. Neonates can produce hepatotoxic metabolites (e.g., NAPQI), but the reduced activity of cytochrome P-450 in neonates may explain the rare occurrence of acetaminophen-induced hepatotoxicity in neonates.
Two European centers have published intravenous dosing guidelines for acetaminophen in neonates [397, 405] and the drug is widely used by anesthetists in the UK. Preliminary data [402, 406, 407] from neonates suggest that current dosing regimens are safe. It must be stressed, however, that these are preliminary data only. The combined subject numbers were only 239 neonates. These numbers are too few to exclude the possibility of future hepatotoxicity; caution and continued monitoring of neonates given acetaminophen are vital. The two studies had daily doses that varied by a factor of two. Both authors report satisfactory analgesia, and so it is probably safer to recommend the smaller rather than the larger dosing regimen. Clearance variability and the lack of a suitable marker of reduced clearance in the first few days after birth also support a recommendation of the smaller dose [403]. Several reports have noted accidental 10-fold overdoses of acetaminophen to young infants that fortunately resolved without sequelae [408, 409, 748]. Extreme care must be taken when administering this medication to neonates.
Nonsteroidal Anti-Inflammatory Drugs
Mechanism of Action
The nonsteroidal anti-inflammatory drugs (NSAIDs) are a heterogeneous group of compounds that share common antipyretic, analgesic and anti-inflammatory effects. NSAIDs act by reducing prostaglandin biosynthesis through inhibition of cyclooxygenase (COX) site of the PGHS enzyme. The prostanoids produced by the COX-1 isoenzyme protect the gastric mucosa, regulate renal blood flow, and induce platelet aggregation. NSAID-induced gastrointestinal toxicity, for example, is likely mediated through blockade of COX-1 activity, whereas the anti-inflammatory effects of NSAIDs are likely mediated primarily through inhibition of the inducible isoform, COX-2.
Pharmacodynamics
The NSAIDs are commonly used in children for antipyresis and analgesia. The anti-inflammatory properties of the NSAIDs have, in addition, been used in such diverse disorders as juvenile idiopathic arthritis (JIA), renal and biliary colic, dysmenorrhea, Kawasaki disease, and cystic fibrosis. The NSAIDs indomethacin and ibuprofen are also used to treat delayed closure of patent ductus arteriosus (PDA) in preterm infants.
There are no linked PK–PD studies of NSAID analgesia or fever in neonates or infants. Investigation of a concentration-response relationship for PDA closure shows that a target serum concentration of 3.5 mg/L is associated with 90 % PDA closure. Dosing increases with PMA to achieve this target because clearance increases with age [410].
NSAID-associated analgesia has been compared to analgesia from other analgesics or analgesic modalities, e.g., caudal blockade, acetaminophen or morphine in children. These data confirm that NSAIDs in children are effective analgesic drugs, improving the quality of analgesia, but they do not quantify the effect. It is not possible to develop an understanding of the dose-effect relationship from these data, nor is it possible to determine if equipotent doses are being compared. The onset of analgesia may correlate with CSF concentrations. CSF penetration after ketorolac, diclofenac, ibuprofen, and indomethacin is rapid in children with peak concentrations reached approximately 30 min after IV administration of routine doses [411–414].
Pharmacokinetics
NSAIDs are rapidly absorbed in the gastrointestinal tract after oral administration in children. The relative bioavailability of oral preparations approaches unity. The rate and extent of absorption after rectal administration of NSAIDs such as ibuprofen, diclofenac, flurbiprofen, indomethacin and nimesulide are less than after the oral routes.
The apparent volume of distribution is small in adults (<0.2 L/kg) but larger in children. The volume of distribution in preterm neonates (22–31 weeks of gestational age) given IV ibuprofen was 0.62 (S.D. 0.04) L/kg [415]. The ibuprofen central volume decreased dramatically after closure of the PDA in preterm neonates (0.244 vs 0.171 L/kg) [416]. The NSAIDs, as a group, are weakly acidic, lipophilic and highly protein bound. The bound fraction is larger in preterm neonates and children, but less than in adults. The impact of altered protein binding is probably minimal with routine dosing because NSAIDs cleared by the liver have a low hepatic extraction ratio [417].
NSAIDs undergo extensive phase I and phase II enzyme biotransformation in the liver, with subsequent excretion into urine or bile. Renal elimination is not an important elimination pathway for the commonly used NSAIDs. Pharmacokinetic parameter estimate variability is large, in part, attributable to covariate effects of age, size, and pharmacogenomics. Ibuprofen, for example, is metabolized by the CYP2C9 and CYP2C8 subfamily. Considerable variation exists in the expression of CYP2C activities among individuals, and functional polymorphism of the gene coding for CYP2C9 has been described [418]. CYP2C9 activity is low immediately after birth (21 % of adult), subsequently increasing progressively to reach a peak activity within 3 months, when expressed as mg/kg/h [52, 419]. Clearance (L/h/kg) is generally greater in children than it is in adults, as we might expect when the linear per kilogram model is used. Ibuprofen clearance increases from 2.06 mL/h/kg at 22–31 weeks’ PCA [415] and 9.49 mL/h/kg at 28 weeks’ PCA [416] to 140 mL/kg/min at 5 years [420]. Similar data exist for indomethacin [421–423].
Many NSAIDs exhibit stereoselectivity [424]. Ibuprofen stereoselectivity is reported in preterm neonates (<28-week gestation). R- and S-ibuprofen half-lives were about 10 h and 25.5 h, respectively. The mean clearance of R-ibuprofen (12.7 mL/h) was about 2.5-fold greater than for S-ibuprofen (5.0 mL/h) [425].
During pregnancy, there is relatively little transfer of NSAIDs from maternal to fetal blood. Very small quantities of NSAIDs are secreted into breast milk. Similarly, infant exposure to ketorolac via breast milk is estimated to be only 0.4 % of maternal exposure [426].
Drug Interactions
NSAIDs undergo drug interactions through altered clearance and competition for active renal tubular secretion with other organic acids. A large fractional protein binding has been proposed to explain drug interactions between NSAIDs and oral anticoagulant agents, oral hypoglycemics, sulphonamides, bilirubin and other protein-bound drugs. An influential paper by Aggeler et al. [427] showed that warfarin administered with phenylbutazone increased both the plasma warfarin concentrations and the prothrombin time in normal volunteers. Phenylbutazone displaces warfarin from its albumin binding sites in vitro, but this observation should not be extrapolated to explain changes in prothrombin time. The observed effect is due to changes in competition for similar drug metabolic clearance pathways and not from changes in protein binding [417].
Adverse Effects
NSAIDs have the potential to cause gastrointestinal irritation, blood clotting disorders, renal impairment, neutrophil dysfunction and bronchoconstriction; effects are attributed to COX-1/COX-2 ratios, although this concept may be an oversimplification.
Ibuprofen reduces the GFR by 20 % in preterm neonates, affecting aminoglycoside clearance, an effect that appears to be independent of gestational age [90]. No significant difference in the change in cerebral blood volume, change in cerebral blood flow or tissue oxygenation index was found between administration of ibuprofen and placebo in neonates [428].
The risk of acute GI bleeding in children given short-term ibuprofen was estimated to be 7.2/100,000 (CI 2–18/100,000) [429, 430], a prevalence not different from children given acetaminophen. The incidence of clinically significant gastropathy in children given NSAIDs for JIA is comparable to that in adults given long-term NSAIDs [431, 432], but the prevalence of gastroduodenal injury may be very much greater depending on the assessment criteria (e.g., abdominal pain, anemia, endoscopy) applied. Data for neonates are not available.
The commonly used NSAIDs have reversible antiplatelet effects, which are attributable to the inhibition of thromboxane synthesis. Bleeding time is usually slightly increased, but remains within normal limits in children with normal coagulation systems. Neonates given prophylactic ibuprofen to induce PDA closure did not have an increased frequency of intraventricular hemorrhage [433].
Opioid Analgesic Drugs
Morphine
Morphine is the most commonly used opioid in neonates and infants. Morphine’s main analgesic effect is by supraspinal μ1-receptor activation. The μ2-receptor in the spinal cord plays an important analgesic role when the drug is administered by the intrathecal or epidural route [434]. Morphine is soluble in water, but lipid solubility is poor compared with other opioids. Morphine’s low oil/water partition coefficient of 1.4 and its pKa of 8 (10–20 % unionized drug at physiologic pH) contribute to delayed onset of peak action with slow CNS penetration.
Pharmacodynamics
Target analgesic plasma concentrations are believed to be 10–20 ng/mL after major surgery in neonates and infants [71, 435]. The concentration required for sedation during mechanical ventilation may be greater (125 ng/mL) [436]. The large pharmacokinetic and pharmacodynamic variability requires that morphine is often titrated to effect using small incremental doses (0.02 mg/kg) in neonates and infants suffering postoperative pain [437]. The effect compartment equilibration half-time (T 1/2keo) for morphine is ~17 min in adults [438] and is estimated to be 8 min in the full-term neonate [439]. The role of morphine for nursing procedures in the neonatal unit remains controversial. Morphine did not reduce pain responses during endotracheal suctioning, despite plasma concentrations up to 400 ng/mL [64]. Intermittent intravenous acetaminophen reduces the morphine requirements in neonates after major surgery [396].
The principle metabolites of morphine, morphine-3-glucuronide (M3G) and morphine-6-glucuronide (M6G), have pharmacologic activity. Contributions to both the desired effect (analgesia) and the undesired effects (nausea, respiratory depression) of M6G are the subject of clinical controversy [440]. It has been suggested that M3G antagonizes morphine and contributes to the development of tolerance [441].
Opioid analgesics including morphine are widely used to control painful responses in neonates. However, evidence from animals suggests that their use may cause long-term adverse sequelae. In humans, the neonatal use of morphine exerted no long-term effects measured in children 8–9 years of age in terms of intelligence, behavior and visual motor function [442].
Pharmacokinetics
Morphine is primarily metabolized by the hepatic enzyme UGT2B7 into M3G and M6G. Sulphation and renal clearance are minor pathways in adults but are more dominant in neonates. Clearance is perfusion limited with a high hepatic extraction ratio. Oral bioavailability is ~35 % due to this first pass effect. The metabolites are cleared by the kidney and, in part, by biliary excretion. Impaired renal function leads to M3G and M6G accumulation.
Morphine clearance matures with PMA reaching adult values at 6–12 months [64]. Clearance increases from 3.2 L/h/70 kg at 24 weeks’ PMA to 9.3 L/h/70 kg at 32 weeks’ PMA and 19 L/h/70 kg at term (Fig. 3.7). The volume of distribution is increased in preterm ventilated neonates (190 L/70 kg) compared with full-term neonates whose lungs were not ventilated (122 L/70 kg) [64]. The volume of distribution in term neonates increased from 83 L/70 kg at birth to a mature value of 136 L/70 kg within 3 months. Metabolite (M3G, M6G) clearance increases with age similar to that described for GFR maturation in infants [63] (Fig. 3.17).


Fig. 3.17
Morphine administered as a bolus 0.07 mg/kg with subsequent infusion 0.03 mg/kg/h in a neonate (a) and a 1-year-old child (b). The concentrations of parent drug and metabolites are different in the two individuals because of the complex interplays between formation and elimination clearances. (Data from Bouwmeester NJ, Anderson BJ, Tibboel D, Holford NH. Developmental pharmacokinetics of morphine and its metabolites in neonates, infants and young children. Br J Anaesth. 2004;92:208–17) [63]
Although an infusion of 5–10 mcg/kg/h will achieve a target concentration of 10 ng/mL in a typical term neonate, clearance is affected by PMA and pathology [65, 443]. Recent evidence indicates that morphine infusions of 4-5 mcg/kg/h provides effective analgesia with minimal risk of overdose in neonates <10 days PNA whereas morphine infusions of 11–23 mcg/kg/h provide reasonable analgesia in older neonates and infants [444]. Morphine pharmacokinetic parameters show large interindividual variability contributing to the range of morphine serum concentrations observed during constant infusions. Protein binding of morphine is small in preterm neonates and has minimal impact on the disposition changes with age. The M6G/morphine ratio also changes with age (Fig. 3.18) because of desynchrony between the maturation of metabolite formation and elimination pathways, but the clinical effect of these ratio changes is unclear.


Fig. 3.18
Composite Emax model, showing hyper- and hypotensive effect of dexmedetomidine on mean arterial blood pressure. From Potts [623]
Although morphine is usually administered intravenously to neonates, other routes have been used. A large variability in the analgesic effect of morphine has been observed after rectal administration; this is a major disadvantage of this route. Although this route is commonly used [445], delayed absorption with multiple doses causing respiratory arrest has been reported [446]. Morphine (25–50 mcg/kg) can also be given via the caudal route to neonates [447, 448]. While systemic absorption is slow, morphine spreads within the CSF to the brainstem where it may cause respiratory depression lasting from 6 h to >18 h [449].
Adverse Effects
Respiratory depression may occur at concentrations of 20 ng/mL [42] in infants and children, but concentration-response relationships in neonates, particularly preterm neonates prone to physiological apnea, are unknown. Hypotension, bradycardia and flushing reflect morphine’s histamine-releasing characteristics. These are more pronounced with rapid intravenous bolus administration [450, 451]. The incidence of vomiting in postoperative children is related to the morphine dose. Morphine doses in excess of 0.1 mg/kg are associated with a >50 % incidence of vomiting in infants and children [398, 452, 453].
It is difficult to quantify this adverse effect in awake healthy neonates who commonly regurgitate after feeding.
Withdrawal symptoms may be observed in neonates after cessation of a continuous morphine infusion for >2 weeks and after infusion periods <2 weeks if the morphine infusion rate is >40 μg/kg/h. Strategies to prevent withdrawal from morphine include the use of neuraxial analgesia, nurse-controlled sedation management protocols, ketamine or naloxone mixed with morphine infusion and alternate agents (e.g., methadone) with lower potential for tolerance [454, 455].
Fentanyl
Fentanyl offers greater hemodynamic stability than morphine, a rapid onset (T 1/2keo = 6.6 min in adults) a short duration of effect. Its relative increased lipid solubility and small molecular conformation enables efficient penetration of the BBB and redistribution.
Pharmacodynamics
Fentanyl is a potent μ-receptor agonist with a potency 70–125 times greater than that of morphine. A plasma concentration of 15–30 ng/mL is required to provide total intravenous anesthesia (TIVA) in adults, whereas the EC50, based on EEG evidence is 10 ng/mL [456, 457]. Fentanyl has been shown to effectively prevent preterm neonates from surgical stress responses and to improve postoperative outcome [458]. Single doses of fentanyl (3 mcg/kg) can reduce the physiologic and behavioral measures of pain and stress associated with mechanical ventilation in preterm infants [459]. Fentanyl has similar respiratory depression in infants and adults when plasma concentrations are similar [460].
Pharmacokinetics
Fentanyl is metabolized by oxidative N-dealkylation (CYP3A4) into nor-fentanyl and hydroxylized. All metabolites are inactive and a small amount of fentanyl is eliminated via the kidneys unchanged. Fentanyl clearance is 70–80 % of adult values in term neonates and, when standardized to a 70-kg person, reaches adult values (approx. 50 L/h/70 kg) within the first 2 weeks of life [35]. Clearance matures with gestational age: 7 ml/min/kg at 25 weeks’ PMA, 10 ml/min/kg at 30 weeks’ PMA and 12 ml/min/kg at 35 weeks’ PMA [461]. Fentanyl’s volume of distribution at steady state (Vss) is ~5.9 L/kg in term neonates and decreases with age to 4.5 L/kg during infancy, 3.1 L/kg during childhood and 1.6 L/kg in adults [30]. This increased Vss results in a smaller blood concentration after bolus administration in neonates and infants. Administration of fentanyl 3 mcg/kg in infants intraoperatively neither depressed respiration nor caused hypoxemia in a placebo-controlled trial [462, 463].
Fentanyl clearance may be impaired with decreased hepatic blood flow, e.g., from increased intra-abdominal pressure in neonatal omphalocele repair [464]. Infants with cyanotic heart disease had reduced Vss and greater plasma concentrations of fentanyl with infusion therapy [465]. These greater plasma concentrations resulted from a reduced clearance (34 L/h/70 kg) that was attributed to hemodynamic disturbance and consequent reduced hepatic blood flow [466].
Fentanyl is widely distributed with a short duration of effect as a result of redistribution to deep, lipid-rich compartments. Fentanyl redistributes slowly from lipid-rich tissues after discontinuation of therapy, resulting in prolonged periods of sedation and respiratory depression. Although CYP3A4 is subject to single nuclear polymorphisms with slow and rapid metabolizers, redistribution rather than clearance is responsible for offset of the drug effect after single-dose therapy. The context-sensitive half-time (CSHT) after a 1 h infusion of fentanyl is ~20 min, which increases to 270 min after an 8-h infusion in adults [467]. While the CSHT is reduced in children [468], there are no data in neonates. Alternative administration routes (e.g., transdermal and transmucosal) have not been studied in neonates [469]. Epidural fentanyl is widely combined with an amide local anesthetic for provision of postoperative analgesia, although there is limited evidence to support such a combination in young children when a therapeutic concentration of local anesthetic is administered [470]. Spread beyond the level of administration is dose dependent, but limited and respiratory depression is uncommon [471, 472].
Adverse Effects
Neonatal tolerance to synthetic opioids develops more rapidly (3–5 days) than it does with morphine (2 weeks) and diamorphine (>2 weeks) [473]. Fentanyl also has a propensity for muscular rigidity and laryngospasm with doses as little as 2 mcg/kg IV in neonates [474, 475]. Other drugs metabolized by CYP3A4 (e.g., cyclosporin, erythromycin) may compete for clearance and increase fentanyl plasma concentrations.
Respiratory depression (hours) may outlast fentanyl’s analgesic effect (35–45 min) due to the prolonged CSHT and/or recirculation of fentanyl bound to the stomach’s acid medium (up to 20 % of an IV dose) or delayed release from peripheral compartments.
Remifentanil
Remifentanil resembles fentanyl, sufentanil, and alfentanil in chemical structure. It is a selective μ-receptor agonist with a greater potency than alfentanil. Its brief elimination half-life of 3–6 min [77, 476] means it is usually given as an infusion [477, 478]. Intravenous remifentanil doses of 0.25 μg/kg/min appear to be safe and effective in neonates [479, 480].
Pharmacodynamics
A target plasma concentration of 2–3 mcg/L is adequate for laryngoscopy and 6–8 mcg/L for laparotomy, and 10–12 mcg/L might be sought to ablate the stress response associated with cardiac surgery [481]. Analgesic concentrations are 0.2–0.4 mcg/L. The T 1/2keo is 1.16 min in adults [76], but the neonatal T 1/2keo has not been reported. Analgesic alternatives should be available for when the short-duration analgesic effect from remifentanil has dissipated. Reports of a rapid development of μ-receptor tolerance with remifentanil use are conflicting. Activity at δ-opioid receptors may contribute [482].
Pharmacokinetics
Remifentanil is metabolized by nonspecific esterases in tissues and erythrocytes to carbonic acid [483, 484]; these esterases appear to be mature even in preterm neonates [74]. Carbonic acid is excreted through the kidneys. Metabolism is independent of liver and renal functions. Clearance in patients with butyryl-cholinesterase deficiency is unaffected.
Remifentanil clearance can be described in all age groups by simple application of an allometric model [485]. This standardized clearance of 2,790 mL/min/70 kg is similar to that reported by others in children [77, 486] and adults [76, 483]. The smaller the child, the greater the clearance when expressed as mL/min/kg. Clearances decrease with age, with rates of 90 mL/kg/min in infants <2 years of age, 60 mL/kg/min in children 2–12 years of age and 40 mL/kg/min in adults [75, 485, 486]. The steady state volume of distribution was greatest in infants <2 months of age (452 mL/kg) and decreased to 308 mL/kg in children 2 months to 2 years and to 240 mL/kg in children >2 years [77]. Elimination half-life appears to be constant, ~3 to 6 min independent of the age of the patient and duration of the infusion [77, 476]. Intravenous remifentanil doses of 0.25 μg/kg/min appear to be safe and effective in neonates [479, 480], but PK–PD data in this age group remain limited.
Although covariate effects such as cardiac surgery appear to have a muted effect on PK, as is seen with propofol, cardiopulmonary bypass (CPB) does have an impact. Remifentanil dosage adjustments are required during and after CPB due to marked changes in its volume of distribution [487]. Other PK changes during CPB are consistent with adult data in which a decreased metabolism occurred with a reduced temperature [488] and with reports of greater clearance after CPB (increased metabolism) compared with during CPB [486].
Adverse Effects
Respiratory depression is concentration dependent [489, 490]. Muscle rigidity remains a concern with bolus doses above 3 mcg/kg used for intubation in neonates [491]. The initial loading dose of remifentanil may cause hypotension and [492] bradycardia prompting some to target the plasma rather than effect site concentration when initiating infusion. This hypotensive response has been quantified in children undergoing cranioplasty surgery. A steady state remifentanil concentration of 14 mcg/L would typically achieve a 30 % decrease in MAP. This concentration is twice that required for laparotomy but is easily achieved with a bolus injection. The T 1/2keo of 0.86 min for this hemodynamic effect [493] is less than remifentanil-induced spectral edge changes described in adults (T 1/2keo = 1.34 min) [76, 494]. Neonatal data are very limited.
Because the inhibitory neurotransmitter, glycine, is included in the formulation of remifentanil, it should not be used for spinal or epidural applications [495].
Alfentanil
Alfentanil is a synthetic opioid that is chemically related to fentanyl. It has a rapid onset (T 1/2keo =0.9 min in adults), a brief duration of action and one-fourth the potency of fentanyl. Alfentanil has reduced lipid solubility and causes less histamine release than fentanyl [496]. The analgesic dose of alfentanil for endotracheal intubation and suctioning in preterm neonates is 10–20 μg/kg [497–499]. A target plasma concentration of 400 ng/mL is used in anesthesia. Metabolism is through oxidative N-dealkylation by CYP3A4 and O-dealkylation and then conjugation to end products that are excreted via the kidney [500]. The plasma protein binding of alfentanil increases from 65 % in preterm neonates to 79 % in term infants and then to ~90 % in adults [501, 502]. The volume of distribution (Vss) in children (0.163 L/kg) is one-third that in adults (0.457 L/kg) [503]. Clearance in neonates (20–60 mL/min/70 kg) is one-tenth that in adults (250–500 mL/min/70 kg [698]). In preterm neonates, the half-life is as long as 6–9 h [504, 505]. Alfentanil cannot be used without neuromuscular-blocking drugs in neonates because of the frequency of rigidity [497, 506].
Sufentanil
Sufentanil is 5–10 times more potent than fentanyl, with a T 1/2keo of 6.2 min in adults [507]. A concentration of 5–10 ng/mL is required for TIVA and 0.2–0.4 ng/mL for analgesia. Pharmacodynamic differences are suggested in neonates. The plasma concentration of sufentanil at the time of additional anesthetic supplementation to suppress hemodynamic responses to surgical stimulation was 2.51 ng/mL in neonates, significantly greater than the concentrations of 1.58, 1.53, and 1.56 ng/mL observed in infants, children and adolescents, respectively [508].
Elimination of sufentanil has been suggested by O-demethylation and N-dealkylation in animal studies. Like fentanyl and alfentanil, the P-450 CYP3A4 enzyme is responsible for the N-dealkylation [509]. Clearance in neonates undergoing cardiovascular surgery (6.7 ± 6.1 mL/kg/min) is reduced compared with values of 18.1 ± 2.7, 16.9 ± 3.2 and 13.1 ± 3.6 mL/kg/min in infants, children and adolescents, respectively [508]. Clearance rates in infants (27.5 ± 9.3 mL/kg/min) were greater than those in children (18.1 ± 10.7 mL/kg/min) in another study of children undergoing cardiovascular surgery [510]. Maturation of clearance is rapid [511], and clearance maturation standardized to a 70-kg person using allometry is similar to that of other drugs that depend on CYP3A4 for metabolism (e.g., levobupivacaine, fentanyl, alfentanil) (Fig. 3.7) [512]. The volume of distribution at steady state (Vss) was 4.15 ± 1.0 L/kg in neonates, greater than the values of 2.73 ± 0.5 and 2.75 ± 0.5 L/kg observed in children and adolescents, respectively [508, 513]. Clearance in healthy children (2–8 years) was greater (30.5 ± 8.8 mL/kg/min) than those undergoing cardiac surgery [513]. Decreased hepatic blood flow reduces clearance [513].
The free fraction of sufentanil decreases with increasing age (neonates 19 %, infants 11 %, children and adults 8 %) and is strongly correlated with the alpha 1-acid glycoprotein plasma concentration [501]. The reduced concentration of alpha 1-acid glycoprotein in neonates and infants [514] increases the free fraction of sufentanil in these age groups. Although sufentanil, fentanyl, alfentanil, and remifentanil have >70 % protein binding and have high hepatic (or nonhepatic for remifentanil) extraction ratios, protein binding changes are probably clinically unimportant [515] because the dose is titrated to effect, and variability in the clearance has a much greater impact.
Codeine
Codeine, or methylmorphine, is a morphine-like opioid with 1/10 the potency of morphine. It is mainly metabolized by glucuronidation, but minor pathways are N-demethylation to norcodeine and O-demethylation to morphine. Approximately 10 % of codeine is metabolized to morphine. As the affinity of codeine for opioid receptors is very low, the analgesic effect of codeine is mainly due to its metabolite, morphine [522]. Codeine is effectively a prodrug analgesic. The continued use of this minor opium alkaloid for pediatric analgesia remains baffling [523] and is subject to debate because it is a prodrug [524]. The CYP2D6 enzyme catalyses the metabolism of codeine to morphine. A genetic polymorphism of this enzyme causes distinct phenotypes responsible for the presence of ultrarapid extensive, extensive and slow codeine metabolizers [525–527, 753]. Between 7 % and 10 % of the European population are believed to be slow metabolizers of codeine [526, 528, 529], but this percentage varies with race [526, 528]. In poor metabolizers, codeine confers little or no analgesia, although adverse effects persist [529]. In ultrarapid metabolizers on the other hand, a large incidences of adverse effects might be expected including apnea and death, because of large plasma morphine concentrations [754]. A consensus guideline has been drafted on whether genetic testing for CYP2D6 polymorphisms should be undertaken before patients are prescribed codeine and if they are tested, subsequent managements based on the genetic results [530].
Codeine can be administered by intramuscular, oral and rectal routes. Intravenous codeine is not recommended because of hypotensive effects [531]. Blood concentrations after rectal codeine are less than those after intramuscular codeine because of incomplete, slower, more variable absorption [532]. Codeine is often used in combination with acetaminophen or NSAIDs. The addition of codeine to acetaminophen has been shown to improve postoperative pain relief in infants [533]. The analgesic effect of the combination of acetaminophen (10–15 mg/kg) and codeine (1–1.5 mg/kg) was comparable to that of ibuprofen (5–10 mg/kg) in children after tonsillectomy [534]. Codeine has been used in infants and neonates after major surgery as an adjunct to acetaminophen or NSAIDs (optimal oral codeine dosage of 1–1.5 mg/kg every 4–6 h, oral acetaminophen of 20 mg/kg every 6 h in infants older than 3 months) [535]. It is anticipated that analgesic alternatives should be available for when the short-duration analgesic effect from remifentanil has dissipated, preterm neonates would gain little analgesic benefit from codeine because the conversion rate to morphine is limited. Maturation of CYP2D6 should follow that of M1 production from tramadol (Fig. 3.5), a metabolite also produced by CYP2D6. There is rapid maturation of this enzyme after 40 weeks’ PMA, suggesting that post-term neonates could benefit, although there are no studies investigating this drug in neonates.
The pharmacokinetics of codeine is poorly described in children despite several decades of use. A volume of distribution (V) of 3.6 L/kg and a clearance (CL) of 0.85 L/h have been described in adults, but there are few data detailing the developmental changes in pediatric pharmacokinetics. The neonatal half-life in neonates (4.5 h) is greater than that in the infant (2.6 h) as a result of immature clearance [536]. Administration (especially of codeine preparations with an antihistamine and a decongestant) in the neonate may cause intoxication [537]. A neonate has died from morphine poisoning when his mother used codeine while breastfeeding. The mother, an ultrarapid metabolizer (UM), produced much more morphine when taking codeine than most people do [538, 539]. Further evidence suggests that the combination of an UM of CYP2D6 and decreased expression of the P-glycoprotein gene, ABCB1, which codes for transport of codeine (and other compounds) out of the central nervous system, predicted 87 % of the infant and maternal central nervous system depression to codeine [530]. When guidelines were followed to improve the safety of codeine during breastfeeding, genotyping did not predict neonatal sedation. Rather, neonatal sedation (2.1 %) occurred only when mother’s codeine consumption exceeded the recommended guidelines [540].
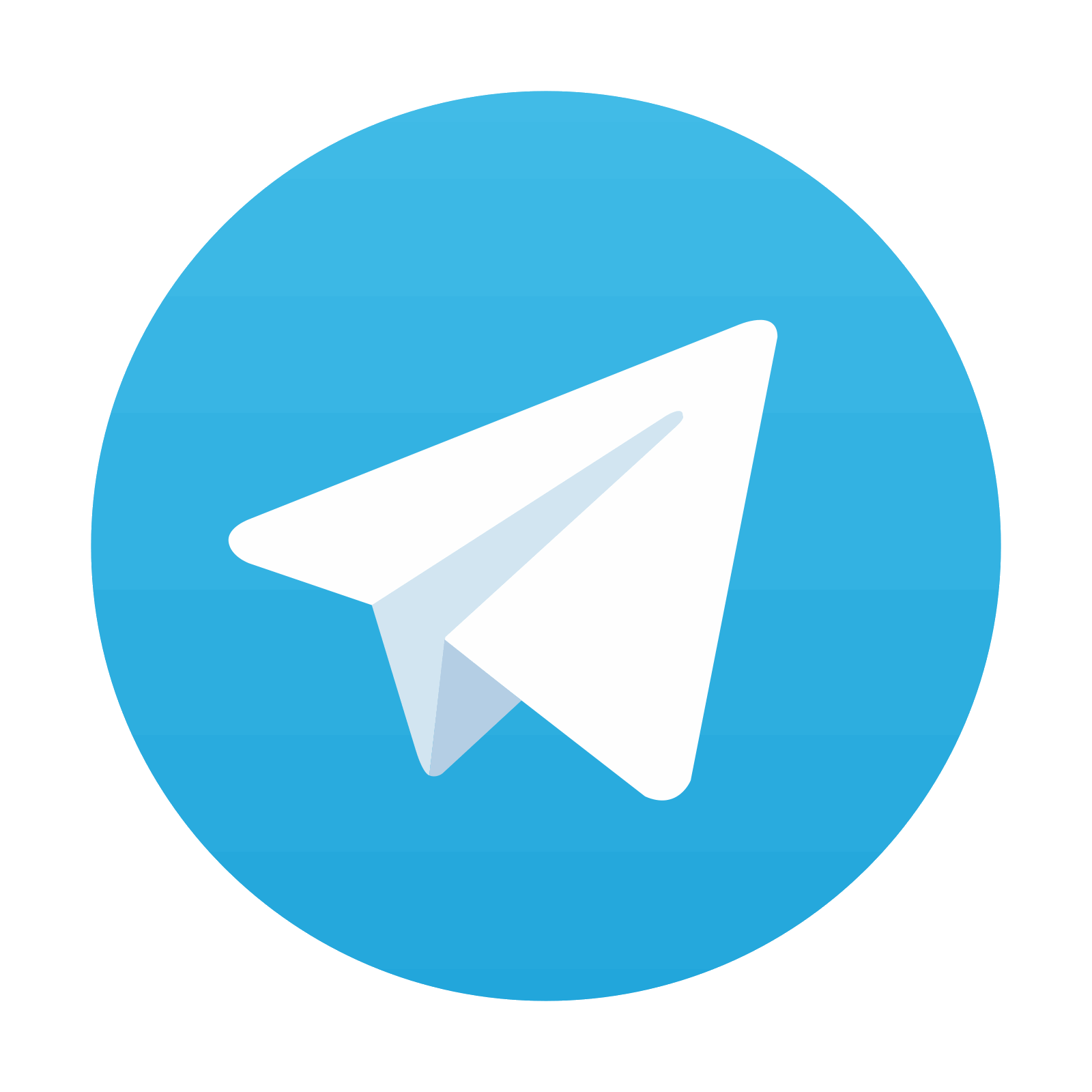
Stay updated, free articles. Join our Telegram channel
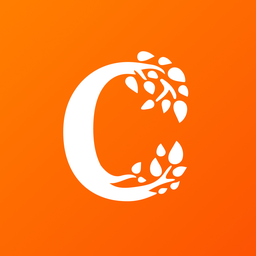
Full access? Get Clinical Tree
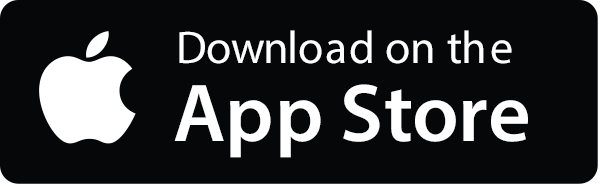
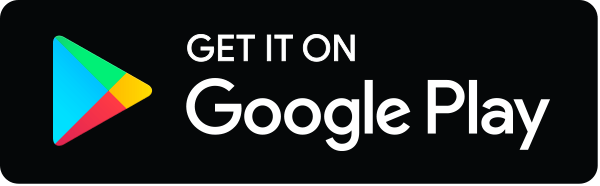