Anatomy of the Neuraxis
Quinn H. Hogan
Anatomy, the oldest of medical sciences, holds a central position in regional anesthesia because of the obvious necessity of correctly delivering the therapeutic solution to the target neural structures. Beyond this, anatomic understanding is required to avoid complications due to needle damage or incorrectly placed injection, to understand the physiologic events resulting from neural blockade, and to develop new techniques for anesthesia and pain treatment. The spinal column, and the soft tissues and neuraxial elements contained within it, have been the subject of examination for hundreds of years, but we do not yet fully understand important aspects of their structure. This is in part due to the special interests of anesthesiologists in such matters as the organization of tissue planes and the permeability of various barriers that regulate distribution of fluids, interests shared by few other physicians and scientists. An additional challenge is the difficulty of observing the contents of the spinal canal, which are enclosed by sturdy bones and ligaments. Removal of these structures disrupts the natural arrangement of the soft tissues within the canal. Only recently have techniques become available for analyzing these features. This chapter anatomically tours the neuraxis, generally from outer to inner structures, and includes new observations wherever possible.
Vertebral Bones
The stacked vertebral bones reveal a segmentation that is otherwise minimally evident in human anatomy. This metameric arrangement into individual vertebrae also dictates that communication of the peripheral nervous system with the spinal cord must be likewise segmented, despite the lack of any organization into subunits within the spinal cord. Typically, there are 7 cervical vertebrae, 12 thoracic, 5 lumbar, 5 sacral, and 4 coccygeal (Fig. 9-1). Common palpable landmarks (Fig. 9-2) may locate particular levels, including the most prominent spinous process (vertebra prominens), which usually indicates the seventh cervical. The seventh thoracic spine is usually opposite the inferior angle of the scapula, and the line connecting the iliac crests (Tuffier line) crosses the vertebral column most often at the L4–L5 disc. However, clinical ability to estimate segmental level from palpable landmarks is often overestimated, and these indices are subject to natural variability in anatomic parameters (Fig. 9-3) (1). For instance, the first thoracic vertebral spine may be more prominent than the seventh cervical. Also, the Tuffier line may cross the vertebral column as high as the L3–L4 disc or as low as the L5–S1 disc (2,3,4,5). For this reason, and because of a variable amount of subcutaneous fat, the accuracy of predicting the vertebral level of needle insertion is about 50% at best when unaided by radiologic imaging. Most often, the error occurs when the clinician selects a level one or two segments higher than the intended level in the lower thoracic and lumbar regions, or selects a space too low in the upper thoracic and cervical region (6,7,8,9). Errors are comparable in the sitting and lying positions, are greater with obese subjects, and are greater using the vertebra prominens as a reference point compared to the Tuffier line (10,11).
The numerical designations of the vertebra may differ between individuals because of anomalous patterns of vertebral segmentation, which are predominantly found in the lumbosacral spine (12). This parallels the phylogenetic stability of mammalian cervical and thoracic segmentation and the marked variability in the number of lumbar vertebrae, even among primates (13). The last lumbar or first sacral vertebrae is often indeterminate in configuration, with fusion of L5 to S1 in 6.2% (sacralization of L5, bilateral in 1.5%), or incomplete fusion of S1 to S2 in 5.3% (lumbarization of S1, bilateral in 4.1%) (12).
Although the vertebrae differ in design at the various levels of the vertebral column, common elements can be defined. Each consists of posterior elements forming a vertebral arch and the body (or soma) anteriorly (Fig. 9-4A). The lumbar vertebral bodies are distinctly narrowed into an hourglass shape, with a diameter 15% less at the middle than at the endplates (14). Stout pedicles arise on the posterolateral aspects of the body and fuse with the platelike laminae to encircle the vertebral foramen. Together, the vertebral foramina of the adjacent vertebrae in sequence form the vertebral (or spinal) canal. In the cervical and lumbar regions (Fig. 9-4B), the canal approaches a triangular shape, but it is circular and smaller in the thoracic vertebrae. The width of the canal measured between the pedicles is narrowest in the thoracic region (about 17 mm), but widens in both the cervical (25 mm) and lumbar regions (about 22 mm at L1 to about 27 mm at L5) (15,16,17,18,19,20). In contrast, the anterior/posterior diameter of the vertebral canal is fairly constant (about 16 mm) throughout the vertebral column (21).
Between the pedicles of each adjacent pair of vertebrae, there remains a gap called the intervertebral foramen (or nerve root canal; Fig. 9-5) that provides a passage between the paravertebral space and the vertebral canal, and conveys the segmental nerves as well as other neural and vascular structures. The transverse process is based at the junction of the pedicle and lamina and passes laterally. The spinous process projects posteriorly from the midline junction of the laminae, is often bifid in the cervical column, and may not lie in the midline at other levels.
The pattern described here varies somewhat in different regions of the vertebral column. Vertebral bodies are relatively massive at the lumbar levels, but less so in the thoracic column and especially in the cervical region. Whereas the transverse processes are attached to the pedicles and laminae in the lumbar and thoracic zones, they take their origin from the vertebral body in the cervical vertebrae. The spinous processes are steeply angled caudally in the thoracic region, but are nearly
perpendicular to the axis of the column in cervical and lumbar vertebrae.
perpendicular to the axis of the column in cervical and lumbar vertebrae.
![]() Figure 9-1. Vertebral column, in lateral view (left) and posterior view (right), illustrating curvatures, lumbar interlaminar spaces, and the sacral hiatus. |
The sacral vertebral column is a special case. In childhood, the sacral vertebrae are connected by cartilage, but progressively fuse into a single structure after puberty. In the adult, only a narrow residue of the sacral discs persists. The fusion of adjacent vertebrae eliminates the intervertebral foramina. Instead, the concave anterior surface of the sacrum features four pairs of large anterior sacral foramina that provide passage from the midline sacral canal for the anterior rami of the upper four sacral nerves. In contrast with their posterior counterparts, which provide an exit for the posterior primary rami at each level, the anterior foramina are unsealed and provide a ready passage for escape of local anesthetic solution injected into the sacral canal. On the posterior surface of the sacrum, there is a median crest with three or more, but commonly four, variably prominent tubercles, representing the sacral spinous processes. Lateral to this crest and medial to the four posterior sacral foramina is the intermediate sacral crest with a row of four tubercles, representing the upper four sacral articular processes. The remnants of the S5 inferior articular processes are free and prominent, and flank the sacral hiatus, constituting the sacral cornua.
The sacrum is the least predictable portion of vertebral anatomy. Specifically, the volume of the adult sacral canal may vary from 12 mL to 65 mL (22). Typically, fusion of the posterior roof of the sacral vertebral canal is complete down to the S5 level, where the sacral hiatus persists as an opening bordered by the sacral cornua on either side and is covered by the thick, fibrous posterior sacrococcygeal ligament (Fig. 9-6). However, either an entire lack of any posterior bony roof or virtually complete closure of the sacral vertebral canal are found in about 8% of subjects (Fig. 9-7) (23). In 5% of adult sacral bones, the anterior–posterior diameter of the canal at the hiatus is 2 mm or less (22), making access by needle placement very difficult or impossible in some subjects. In children, however, the sacral hiatus is predictably open and access is readily performed.
The coccyx is a small triangular bone consisting of three to five fused rudimentary vertebrae; it attaches by means of its upper articular surface to the lower articular surface of the sacrum. It has two prominent coccygeal cornua that abut their sacral counterparts. The bone tends to be angulated forward from the sacrococcygeal junction, with its pelvic surface facing anteriorly and upward.
Joints of the Vertebral Column
The ligamentous system of the vertebral column must bond the adjacent segments adequately while allowing for a degree of bending motion. The joints between the successive vertebral bones (Fig. 9-8) control this motion.
The adjacent vertebral bodies are joined at their endplates by the discs, which are composed of fibrocartilaginous joints with an avascular gelatinous core—the nucleus pulposus—surrounded by a reinforcing annular ligament made of collagenous lamellae. These serve the purposes of bearing weight while permitting flexibility. In the cervical region, the lateral upper edge of the vertebral bodies extends as the uncinate process, which articulates with the body of the next cephalad vertebra as the uncovertebral joint (of Luschka). Arthritic degeneration and expansion of this joint may encroach upon the cervical nerve root canals.
The posterior elements of adjacent vertebrae articulate by true diarthrodial joints, the zygapophyseal (or facet) joints. The inferior articular process projecting caudally overlaps the superior articular process from the next most caudal vertebra. In the cervical and lumbar column, the facet joints are posterior to the transverse processes, whereas the thoracic facets are anterior to the transverse processes. Joint surfaces are angled midway between the axial and coronal planes in the cervical region, but are much more vertically deployed at thoracic levels, and are almost in a coronal plan (24). At lumbar levels, the facet joints are distinctly curved, such that the posterior portion is in the sagittal plane whereas the anterior portion is almost in the coronal plane. The transition from the characteristic thoracic to lumbar type articulation is gradual in half of subjects, with intermediate articular orientation at T11–T12 (25). In others, the change is abrupt, with a coronal superior facet and a curved/sagittal inferior facet on a single vertebra at T12 (29%), T11 (16%), or L1 (0.5%), with frequent asymmetry.
The orientation of the facets guides and constrains movements between the two vertebrae. Specifically, minimal rotation is allowed at lumbar levels because of the sagittally opposing
portions, whereas thoracic facets permit rotation but limit flexion. In the cervical column, movement in all planes is less restricted and includes translation (anterior/posterior sliding) between adjacent vertebrae controlled by the uncinate processes as lateral guide rails (24). Movement in the sagittal plane (flexion and extension) is maximal in the cervical region and at L5–S1. In addition to restricting motion, the facets bear weight in the cervical region (26) and at the lumbosacral joint (27). Lumbar posterior joints may transmit load in part through contact of the tip of the inferior articular process with the lamina of the vertebra below (28).
portions, whereas thoracic facets permit rotation but limit flexion. In the cervical column, movement in all planes is less restricted and includes translation (anterior/posterior sliding) between adjacent vertebrae controlled by the uncinate processes as lateral guide rails (24). Movement in the sagittal plane (flexion and extension) is maximal in the cervical region and at L5–S1. In addition to restricting motion, the facets bear weight in the cervical region (26) and at the lumbosacral joint (27). Lumbar posterior joints may transmit load in part through contact of the tip of the inferior articular process with the lamina of the vertebra below (28).
![]() Figure 9-5. Boundaries of the epidural space. Note that the posterior epidural space at each level has its greatest anterior–posterior dimension at its most superior portion. |
The capsule of the facets is loose and redundant at the inferior and superior ends of the joint, and injection of the joints is often accompanied by leakage of the solution into the epidural space from medial disruption of the capsule (29,30,31). Rudimentary fibroadipose menisci and synovial folds cushion the superior and inferior poles of the lumbar zygapophyseal joints (32), but these typically disappear with age and the cartilage on the joint surfaces thins (33). Although small myelinated nerves innervate the facet menisci (34), no clear evidence implicates them in chronic back pain.
Facet arthritis with periarticular exostoses is another etiology of cord and nerve compression (35), and pathologic medial expansion of facets may interfere with epidural or spinal needle placement as well.
Ligaments of the Vertebral Column
The supraspinous ligament joins the tips of the spinous processes as a heavy band (Fig. 9-8), but thins and vanishes in the lower lumbar region (36), which allows greater flexion
at the L5–S1 joint. Between the spinous processes, the interspinous ligament forms a narrow web. It may have a slit-like midline cavity filled with fat (36), which may mistakenly give a positive loss-of-resistance sign during epidural needle insertion. The supraspinous and intraspinous ligaments are largely composed of collagen so that a needle passing through them generates a characteristic snapping sensation as the fibers are parted. In contrast, the ligamentum flavum is 80% elastin, with a dense and homogenous texture that is readily appreciated as a needle passes through it. In normal posture, the ligamentum flavum is under tension, so that it retracts to half its length when cut. It spans from the anterior surface of the upper lamina of an adjacent pair of vertebrae to the posterior aspect of the lower lamina. The right and left halves meet at an angle of less than 90 degrees, and a gap may be present in the midline (37). Its lateral edges wrap anteriorly around the medial margin of the facet joints, reinforcing the joint capsule. Bone may grow into the margins of the ligamentum flavum even in young individuals, especially at mid and lower thoracic levels (38,39,40,41), which may result from the increased rotatory strains at these levels (40). These fine bone spurs in the ligamentum flavum may impede the progress of a spinal or epidural needle and may be mistaken for fracture fragments on x-ray images.
at the L5–S1 joint. Between the spinous processes, the interspinous ligament forms a narrow web. It may have a slit-like midline cavity filled with fat (36), which may mistakenly give a positive loss-of-resistance sign during epidural needle insertion. The supraspinous and intraspinous ligaments are largely composed of collagen so that a needle passing through them generates a characteristic snapping sensation as the fibers are parted. In contrast, the ligamentum flavum is 80% elastin, with a dense and homogenous texture that is readily appreciated as a needle passes through it. In normal posture, the ligamentum flavum is under tension, so that it retracts to half its length when cut. It spans from the anterior surface of the upper lamina of an adjacent pair of vertebrae to the posterior aspect of the lower lamina. The right and left halves meet at an angle of less than 90 degrees, and a gap may be present in the midline (37). Its lateral edges wrap anteriorly around the medial margin of the facet joints, reinforcing the joint capsule. Bone may grow into the margins of the ligamentum flavum even in young individuals, especially at mid and lower thoracic levels (38,39,40,41), which may result from the increased rotatory strains at these levels (40). These fine bone spurs in the ligamentum flavum may impede the progress of a spinal or epidural needle and may be mistaken for fracture fragments on x-ray images.
![]() Figure 9-8. Ligaments of the lumbar vertebral column, shown in lateral view (A) and sagittal section (B). |
With age, the intervertebral discs become desiccated and lose height, which in turn causes increased overlap of the facets. This decreases the longitudinal dimensions of the intervertebral foramen and foreshortens the ligamentum flavum, causing it to buckle into the foramen, further contributing to foraminal narrowing.
The broad anterior longitudinal ligament reinforces the anterior aspect of the discs and binds the vertebral bodies together. Posterior to the vertebral bodies, the posterior longitudinal ligament likewise constrains the relative motion of the vertebral bodies. It runs along the anterior surface of the dural sac as a tight band, but broadens and merges tightly with the discs at each level (Fig. 9-9). This reinforcement typically prevents herniations of the disc in the midline. At cervical and thoracic levels, ossification may result in spinal stenosis (41).
Epidural Space
Everything outside the dural sac but within the vertebral canal can be considered to constitute the epidural space. Its outer boundaries are thus the walls of the vertebral canal, including the vertebral bodies and discs anteriorly, pedicles laterally, and laminae and ligamenta flava posteriorly. It is commonly said that the epidural space is a “potential space,” but it naturally has contents, specifically fat, vessels, and nerves. There is no clear physiologic purpose in having fat within the spinal canal. Indeed, the cranial epidural space is entirely empty. Whereas the brain is protected by a rigid case, the spinal cord must exist within the flexible vertebral column. Perhaps the epidural fat, which is nearly fluid in texture and which has nonadherent surfaces to permit gliding movement of the neural structures, provides a padding effect.
The distance from the skin to the lumbar epidural space in the midline is on average about 5 cm, but may be as small as 3 cm and rarely greater than 8 cm. Distance loosely correlates with weight, and it is somewhat greater at L3–L4 than at other lumbar levels (42,43,44,45,46,47). A practitioner must be cautious when using these dimensions clinically, however, since substantial variability makes it unreliable to expect the actual depth to be close to the published mean.
The distribution of epidural contents is highly nonuniform. Investigations by cryomicrotome sectioning (Figs. 9-10,9-11,9-12,9-13,9-14) (37) and in vivo imaging avoid the dissection artifact. These methods show that the undisturbed lumbar vertebral canal is nearly filled by the dural sac. Thus, the epidural space is empty in large areas, where the dura contacts bone and ligament. It is important to recognize, however, that the dura is not adherent to the canal wall in these empty areas, and solutions and catheters may pass through them. Separated by these empty areas, the epidural contents occur as a series of metamerically and circumferentially discontinuous compartments (Fig. 9-15). In contrast to this general pattern, the dural sac inferior to the L4–L5 disc and in the sacral canal tapers to a smaller diameter and does not fill the canal as completely,
resulting in a proportionate increase in epidural fat. This may contribute to difficulty in delivering local anesthetic to the L5 and sacral nerve roots during epidural anesthesia, since solution is not confined in close proximity with neural structures at these levels.
resulting in a proportionate increase in epidural fat. This may contribute to difficulty in delivering local anesthetic to the L5 and sacral nerve roots during epidural anesthesia, since solution is not confined in close proximity with neural structures at these levels.
![]() Figure 9-9. Posterior view of the posterior longitudinal ligament, viewed with the posterior vertebral structures removed. |
Because of their distinct designs, the various epidural compartments are considered separately in detail.
Posterior Epidural Compartment
The portion of the epidural space posterior to the dura is filled by a fat pad that is triangular in axial section (Figs. 9-13 and 9-14). (In epidurograms and epiduroscopy, the stretched posterior epidural fat is often mistakenly identified as an epidural fibrous band.) It is enclosed by the ligamenta flava, but also extends slightly under the caudal-most portion of the lamina above (Fig. 9-16). The largest posterior epidural compartment is at the mid-lumbar level, with progressive decrease in anterior–posterior dimension at thoracic levels (Fig. 9-17) (48). Rostral to the C7 level, the posterior epidural space vanishes and the posterior dura lies entirely in contact with the ligamentum flavum and laminar bone (Fig. 9-18). Movement of the dura within the canal during spinal flexion (49,50,51,52) is facilitated by the nonadherent gliding surface of the posterior fat pad.
![]() Figure 9-10. Schematic sagittal section of lumbar vertebrae, indicating the levels of the subsequent figures. Yellow indicates ligamentum flavum, brown indicates bone. |
The cleft-like space between the epidural fat and canal wall (Fig. 9-19) allows passage of catheters and injected fluids, with only a minor impediment in the posterior midline, where the dura may adhere to the lamina or fat (53). This arrangement of apposing nonadherent tissue planes is ideally designed to demonstrate the normal subatmospheric pressure within tissues (54), generated by the usual action of lymphatics and the balance of osmotic and hydrostatic forces across the capillary endothelium (Starling forces). This produces the force that aspirates a hanging drop into the needle hub as the tip enters the compartment. Alternatively, pressurized air or saline enters readily into the plane between the nonadherent posterior fat pad and canal wall at the moment the advancing needle tip passes anterior to the ligamentum flavum, thus signaling entry into the spinal canal by the loss-of-resistance method. Rarely, the needle might pass directly into the substance of the posterior fat pad, allowing a loss of resistance to injection but making catheter passage difficult.
The geometry of the posterior epidural space dictates the distance that a needle must travel after entering the epidural space within the ligamentum flavum before contacting the dura. This dimension is a maximum of about 8 mm at the cephalad extent of the interlaminar space and in the midline (55). However, if the needle enters the spinal canal away from the midline, it may encounter the dura with no further advancement since the posterior epidural fat pad thins laterally and the dura contacts the ligamentum flavum there.
The only point of attachment for this fat is to the posterior midline by a vascular pedicle that enters through the gap between the right and left ligamenta flava (Fig. 9-20).
This mesentery-like attachment and the accompanying fat pad may be seen as a midline filling defect in radiologic contrast studies (56,57,58), and as an incomplete “membrane” during epiduroscopy (59,60). The native posterior epidural compartment is entirely without fibrous tissue (61,62,63), although fibrous proliferation takes place after laminectomy.
This mesentery-like attachment and the accompanying fat pad may be seen as a midline filling defect in radiologic contrast studies (56,57,58), and as an incomplete “membrane” during epiduroscopy (59,60). The native posterior epidural compartment is entirely without fibrous tissue (61,62,63), although fibrous proliferation takes place after laminectomy.
Epidural lipomatosis, in which the posterior epidural fat expands and compresses the dural sac (Fig. 9-21), is well recognized during systemic steroid therapy or endogenous Cushing syndrome and may produce neurologic compromise (64,65). Additionally, symptomatic epidural lipomatosis may occur after epidural steroid treatments (66), associated with morbid obesity (67), or in a normal individual (68). Even the normal posterior epidural fat may contribute to pathology when its displacement by facet arthropathy contributes to central vertebral canal stenosis (69).
Lateral Epidural Compartment
No epidural contents exist lateral to the dural sac where it is in contact with the vertebral pedicles. Between the pedicles, however, the lateral epidural compartment forms just medial to each intervertebral foramen and is filled with segmental nerves, vessels, and fat (Fig. 9-22). Except in extreme cases of degenerative disease, the intervertebral foramina are wide open (Fig. 9-23) and allow the free egress of solution injected within the vertebral canal (53,70). Early observations of a “fibrous operculum” occluding the foramen probably represented an artifact of specimen desiccation. Because of the flexibility of the tissues occupying the lateral epidural space and the lack of a rigid barrier in the intervertebral foramina, the pressure in the epidural space closely reflects abdominal pressure. Increased abdominal pressure, such as happens during a cough or pregnancy, is therefore readily transmitted to the epidural space (71). There is no reason to believe that veins passing through the intervertebral foramina in some way play a special role in conducting pressure changes from the abdomen to the vertebral canal.
Anterior Epidural Space
A fine membrane, sometimes called the fascia of the posterior longitudinal ligament, stretches laterally from the posterior longitudinal ligament and completely separates the anterior epidural compartment from the rest of the vertebral canal (Fig. 9-22). This membrane effectively blocks spread of injected solution from passing anterior to the plane of the posterior longitudinal ligament, and funnels solution toward the spinal nerves
(Fig. 9-24) (70). At the longitudinal level of the narrow midportion of the vertebral body, this anterior space is almost entirely occupied by a nearly confluent internal vertebral plexus, from which the midline basivertebral vein originates as it penetrates into the vertebral body (Figs. 9-25 and 9-26). Catheters that transgress into the anterior epidural space through the fascia of the posterior longitudinal ligament are likely to enter the venous plexus. At the level of each disc, the anterior epidural compartment is obliterated by attachment of the posterior longitudinal ligament to the disc.
(Fig. 9-24) (70). At the longitudinal level of the narrow midportion of the vertebral body, this anterior space is almost entirely occupied by a nearly confluent internal vertebral plexus, from which the midline basivertebral vein originates as it penetrates into the vertebral body (Figs. 9-25 and 9-26). Catheters that transgress into the anterior epidural space through the fascia of the posterior longitudinal ligament are likely to enter the venous plexus. At the level of each disc, the anterior epidural compartment is obliterated by attachment of the posterior longitudinal ligament to the disc.
![]() Figure 9-12. Axial cryomicrotome section (A) and index diagram (B) of a third lumbar vertebra, caudal to the image in Figure 9-11. CE, cauda equina; DRG, dorsal root ganglion; FPLL, fascia of the poster longitudinal ligament; ISL, interspinous ligament; PLL, posterior longitudinal ligament; PSC, perivertebral sympathetic chain; SN, spinal nerve branches; SP, spinous process; SSL, supraspinous ligament; VR, ventral root. Bone is brown, nerves are pink, and veins are blue. |
Functional Implications
Few attachments exist between various structures in the spinal canal. Although dura, fat, nerves, and spinal canal wall are in contact, these tissues are not bound to one another. Therefore, solution injected into the epidural space readily spreads circumferentially at a given level and passes out of the intervertebral foramina (Fig. 9-27), and likewise freely passes longitudinally within the vertebral canal (Fig. 9-28).
As a catheter is advanced through the needle, there may be a brief resistance to advancement as the tip encounters the dura. Computed tomography (CT) shows that catheter tips inserted 3 cm into the vertebral canal most commonly travel laterally to the internal aspect of an intervertebral foramen (Fig. 9-29), because of the stiffness of the fairly short segment of catheter that has emerged from the needle. Nonetheless, injected solutions still surround the dura circumferentially (Fig. 9-30). Even when the catheter tip lies exterior to the intervertebral foramen in the paravertebral space, distribution of the injectate is preferentially back into the vertebral canal. This is explained by high pressures that develop with injection into the muscular confines of the perivertebral space. In contrast, the adjacent spinal canal has a maximum pressure set by the cerebrospinal fluid (CSF) pressure of approximately 15 cm H2O, and therefore accepts flow by displacing CSF. Thus, fluid may arrive in the epidural space during any block around the vertebral column, including brachial plexus blocks (72,73,74), stellate ganglion and lumbar sympathetic chain blocks (75,76), and especially intercostal (77) and paravertebral spinal nerve blocks (78).
Meninges
The spinal cord, nerve roots, and CSF are enclosed in membranes termed meninges (Fig. 9-31).
![]() Figure 9-13. Axial cryomicrotome section (A) and index diagram (B) through the L3–L4 intervertebral disc, caudal to the image in Figure 12. AL, annular ligament; CE, cauda equina; EF, epidural fat; IAP, inferior articular process; NP, nucleus pulposus; PLL, posterior longitudinal ligament; PSC, perivertebral sympathetic chain; SAP, superior articular process; SN, spinal nerve; SP, spinous process; SSL, supraspinous ligament; TS, thecal sac; ZJS, zygapophyseal joint space. Ligamentum flavum is yellow, bone is brown, nerves are pink, and veins are blue. |
Dura
The outermost meningeal layer is the dura mater, a connective tissue sack that extends from the skull, where its fusion with the foramen magnum terminates the epidural space. The caudal tip of the spinal dura is at about the S2 level (Fig. 9-3). The dural sac is attached to the posterior longitudinal ligament, particularly in the lumbar region (79), and is also anchored by the attachment of the anterior aspect of dural nerve root sleeves to the epineural tissue in the intervertebral foramen. The dura develops a posterior midline fold, termed a plica mediana dorsalis, when foreign matter such as injected air or fluid compresses the dural sac (Fig. 9-30B). Under these conditions, scattered attachments of the dura to the posterior epidural fat and lamina produce a tethering effect that tents the dura posteriorly. This is not seen, however, in the undisturbed dura, which is oval in axial section (Figs. 9-12 and 9-13) (37), except where it assumes a triangular shape where the nerve root sheaths diverge laterally (Figs. 9-11 and 9-14). The dura is thickest in the posterior midline and thinner in the lumbar area than more rostral (80). Still, lumbar dura is impenetrable by epidural catheters (81).
The structure of the dura is composed of collagenous lamellae and some elastin elements, separated by clefts filled with ground substance (82), which account for dural permeability (83). Although the fibrous strands run in both circumferential and longitudinal fashion (82), the predominant direction is lengthwise (84). Dura is somewhat elastic, especially in the circumferential dimension (84). Because the dura is freely compressible, shifts in CSF from the intracranial space or between different sections of the vertebral dural sac are reflected as dynamic changes in shape and volume of the sac. For instance, the Valsalva maneuver dramatically and immediately collapses the lumbar and thoracic dural sac, displacing CSF rostrally (85,86,87,88). The displaced CSF distends the cervical dural sac, but does not enter the skull, which is a fixed space and is pressurized comparable to the thoracoabdominal cavity. Tensing of the dura with flexion of the vertebral column, especially at cervical levels, causes an increased CSF pressure (89) and a rostral shift of the dural sac within the vertebral canal of close to 2 cm (49,50,51,52).
Arachnoid and Pia
Immediately within the dura is the arachnoid mater, a thin membrane that encloses the subarachnoid space and the CSF. Delicate trabeculae span the subarachnoid space from the
thecal sac to the pia mater that envelops the surface of the cord and nerve roots (Fig. 9-32). (Because of the intimate relationship, common embryologic origin, and similar microscopic structure of the inner two meninges, they are often designated jointly as pia-arachnoid or leptomeninges.) The basal lamina and tight intracellular junctions of the arachnoid cells, together with those of the pia mater, constitute a physiologically active barrier (20). Whereas the substantia dura can be cleanly punctured, the arachnoid is velamentous and filmy in texture and withdraws from an advancing needle. In doing so, it may delaminate between its component layers (90), forming the commonly termed subdural space. This is probably a misnomer, since the cleft that most easily forms when arachnoid is pulled away from dura is not between the dura and arachnoid membranes, but more properly between layers of arachnoid (91). Conventional radiographic imaging is not adequate to distinguish between fluid layering inside or outside the dura. Secondary indicators have been used, such as lack of flow of contrast along exiting spinal nerves and a cylindrical pattern of uniform layering. However, these have not been suitably validated. For instance, a study of intentional subdural injection shows that solution preferentially passes out along the nerve roots (92), and routine epidural injections may also layer cylindrically outside the dura. A dural-based loculation on CT does, however, prove a subdural injection.
thecal sac to the pia mater that envelops the surface of the cord and nerve roots (Fig. 9-32). (Because of the intimate relationship, common embryologic origin, and similar microscopic structure of the inner two meninges, they are often designated jointly as pia-arachnoid or leptomeninges.) The basal lamina and tight intracellular junctions of the arachnoid cells, together with those of the pia mater, constitute a physiologically active barrier (20). Whereas the substantia dura can be cleanly punctured, the arachnoid is velamentous and filmy in texture and withdraws from an advancing needle. In doing so, it may delaminate between its component layers (90), forming the commonly termed subdural space. This is probably a misnomer, since the cleft that most easily forms when arachnoid is pulled away from dura is not between the dura and arachnoid membranes, but more properly between layers of arachnoid (91). Conventional radiographic imaging is not adequate to distinguish between fluid layering inside or outside the dura. Secondary indicators have been used, such as lack of flow of contrast along exiting spinal nerves and a cylindrical pattern of uniform layering. However, these have not been suitably validated. For instance, a study of intentional subdural injection shows that solution preferentially passes out along the nerve roots (92), and routine epidural injections may also layer cylindrically outside the dura. A dural-based loculation on CT does, however, prove a subdural injection.
![]() Figure 9-14. Axial cryomicrotome section (A) and index diagram (B) of a fourth lumbar vertebra, caudal to the image in Figure 13. CE, cauda equina; IAP, inferior articular process; PLL, posterior longitudinal ligament; PSC, perivertebral sympathetic chain; SAP, superior articular process; SN, spinal nerve branches; SP, spinous process; SSL, supraspinous ligament; TS, thecal sac; ZJS, zygapophyseal joint space. Bone is brown, nerves are pink, and veins are blue. |
Cerebrospinal Fluid
About 500 mL of CSF is formed each day (93,94) principally by the choroid plexuses of the cerebral ventricles (95,96). Bulk flow distributes the fluid into the cisterns at the base of the brain, from which most CSF flows along the convexities of the brain (Fig. 9-32). Along the sagittal sinus, macroscopic defects in the dura accommodate outward herniations of arachnoid membrane, termed arachnoid granulations or Pacchionian bodies. These permit passage of CSF, and probably account for much of the CSF absorption back into the venous circulation, although other mechanisms participate (94). Particulate matter as large as 7 μm may pass through the granulations from CSF into venous blood (97), and electron microscopy has confirmed widened intracellular spaces (98) and transcellular fenestrations (99) in arachnoid granulations. A one-way valve mechanism, by which these passages open only when exposed to a CSF pressure in excess of the venous pressure, assures that flow will only be from CSF to veins. The pressure-sensing feature of arachnoid granulations regulates CSF pressure to about 10 to 20 cm H20 in the lateral position (100).
The bulk of the CSF remains in the skull, whereas a minority of CSF produced leaves the cranial cavity and enters the spinal subarachnoid space to pass downward posterior to the cord
and return upward anterior to the cord (Fig. 9-33). Superimposed upon this bulk flow is a longitudinal oscillation of the CSF column in synchrony with the pulsations of the arteries in the skull (101). The amplitude of this movement is substantial, about 9 mm per cycle in the cervical CSF and about 4 mm at the thoracolumbar junction. It is likely that this oscillatory CSF pulsation facilitates anesthetic distribution after subarachnoid injection. This could in part explain how material injected into the lumbar CSF ascends to the basal cisterns within an hour (102).
and return upward anterior to the cord (Fig. 9-33). Superimposed upon this bulk flow is a longitudinal oscillation of the CSF column in synchrony with the pulsations of the arteries in the skull (101). The amplitude of this movement is substantial, about 9 mm per cycle in the cervical CSF and about 4 mm at the thoracolumbar junction. It is likely that this oscillatory CSF pulsation facilitates anesthetic distribution after subarachnoid injection. This could in part explain how material injected into the lumbar CSF ascends to the basal cisterns within an hour (102).
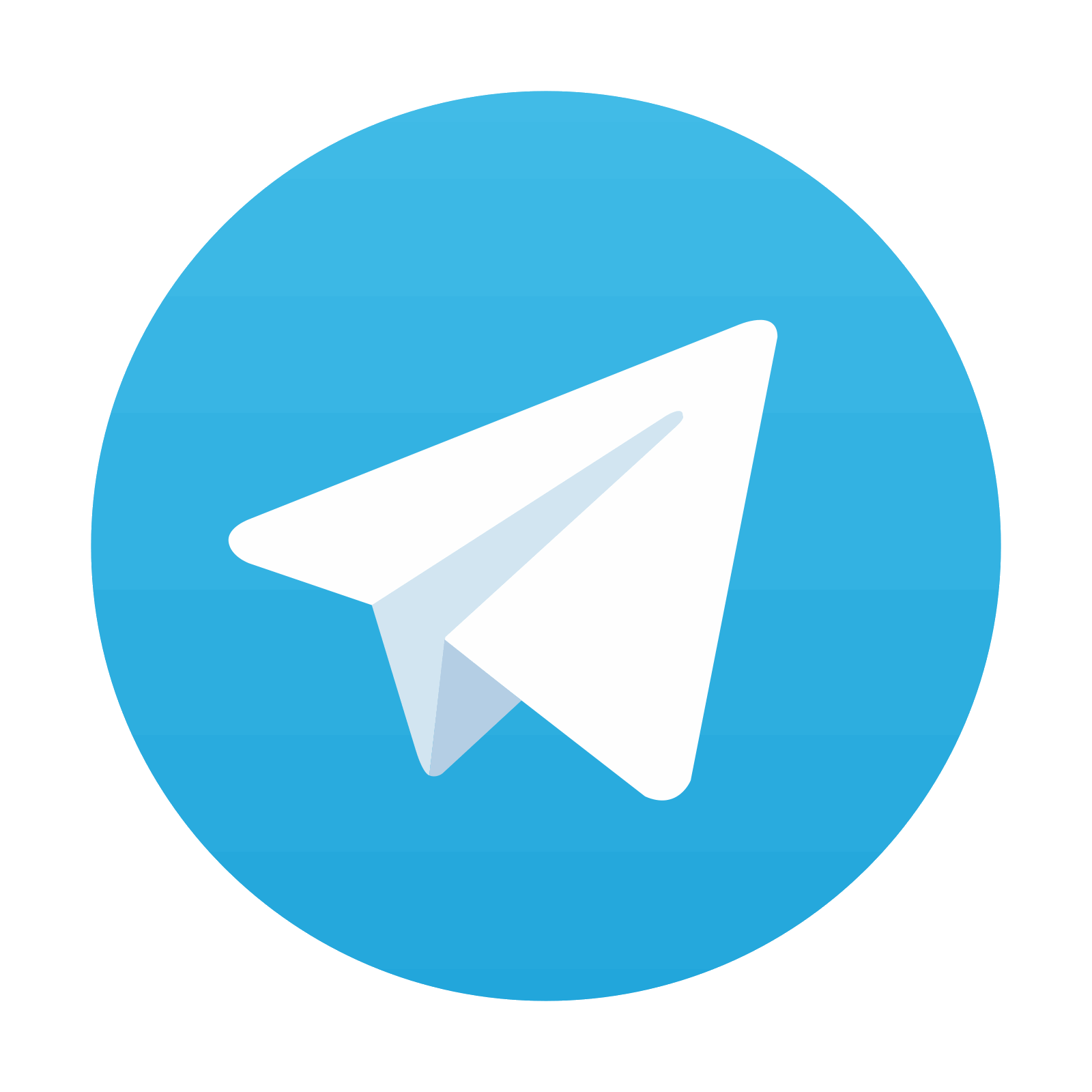
Stay updated, free articles. Join our Telegram channel
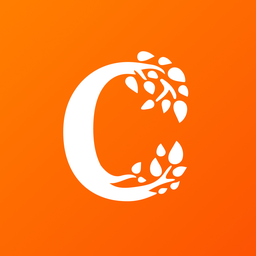
Full access? Get Clinical Tree
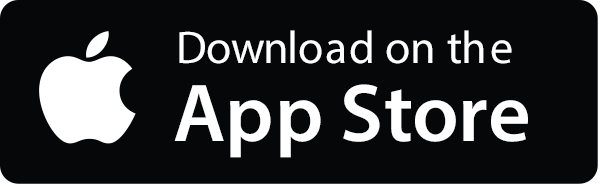
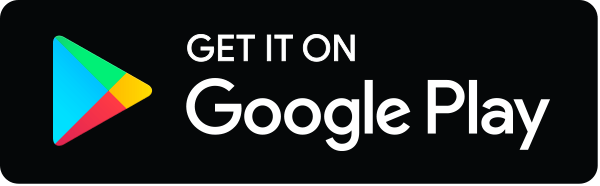