Abstract
The anatomy of the spine is complex and includes the osseus spinal column, joints, spinal canal, intervertebral formina, spinal cord, nerve roots, and spinal vasculature. Back pain may arise from among a variety of etiologies and structures in the spine. Imaging of the spine is an invaluable tool in diagnosing the source of the patient’s pain complaint and pinpointing the exact level of spinal pathology. Diagnostic imaging of back pain is usually accomplished with a combination of spinal radiography, myelography, computed tomography (CT), and magnetic resonance imaging (MRI) tailored towards the patient’s symptoms and localization of pain. A clear understanding of spinal anatomy, common degenerative abnormalities, pain generators, and their typical appearance on imaging forms the necessary basis for treatment of back pain.
Keywords
anatomy, computed tomography, imaging, magnetic resonance imaging, pain, radiology, spine
Acknowledgments
The authors would like to thank Rita Jarmon and David Botos for help in preparing this chapter.
Anatomy
Osseous Spinal Column
The spinal column is typically composed of 7 cervical, 12 thoracic, 5 lumbar, and 5 fused sacral segments. The terminal portion of the osseous spinal column, the coccygeal segments, varies in number, but four segments can typically be visualized. The morphology of the individual vertebrae is quite consistent throughout, except for the first two cervical segments (C1 and C2) and the sacrococcygeal levels.
The C1 level, commonly referred to as the atlas, comprises an anterior arch, posterior arch, and paired lateral masses ( Fig. 9.1A ). The lateral masses articulate with the occipital condyles superiorly and the body of C2 inferiorly (see Fig. 9.1B ). C1 does not have a vertebral body, and it is not separated from adjacent levels by an intervertebral disc. The C2 vertebra, commonly referred to as the axis, has some of the typical features of the remainder of the vertebral segments but is unique in having a superior extension of bone from the vertebral body that articulates with the dorsal margin of the anterior arch of C1: This bony projection is called the odontoid process or dens and allows for head rotation (see Fig. 9.1B ). Unique to the segments from C3 through C7 are the uncinate processes that arise from the dorsolateral margins of the superior end plates of the vertebral bodies and articulate with the level above ( Fig. 9.2 ).


The typical cervical, thoracic, and lumbar vertebrae consist of an anterior body, paired pedicles, articular pillars and laminae, and a single dorsal midline spinous process ( Fig. 9.3 ). The pedicles attach the body to the posterior neural elements. The articular pillars are composed of the pars interarticularis and the superior and inferior articular processes. Each level from C3 to L5 has superior and inferior articular processes that serve as the main posterior contact between adjacent levels. The surface of the superior articular process is the inferior facet of the associated zygapophyseal joint, and the surface of the inferior articular process is the superior facet of the joint. The “superior processes” at C1 and C2 and the “inferior process” at C1 are more descriptively referred to as articular surfaces, as they do not have a true morphologic extension away from the vertebral segments. The two laminae extend dorsomedially and connect to form the root of the spinous process. The spinous process projects dorsally and serves as an attachment point for the posterior ligamentous structures. The pedicles, articular pillars, and lamina serve to enclose and protect the spinal canal and contents, particularly the spinal cord and nerve roots. Transverse processes vary in size from short in the cervical spine to long in the lumbar spine. In the mid-cervical spine, the transverse processes help to enclose and form the osseous transverse foramina that transmit the vertebral artery and contents. In the thoracic and lumbar spine, the transverse processes serve as anchoring points for the muscles that help to stabilize and protect the spinal column and its contents.

Joints
Six specific types of synovial joints exist from the skull base to the lumbosacral junction, including the atlanto-occipital, atlantoaxial, uncovertebral, costovertebral, costotransverse, and zygapophyseal (facet) joints. The atlanto-occipital joint is formed by the bilateral inferiorly convex occipital condyles and the bilateral concave superior articular surfaces of the C1 lateral masses (see Fig. 9.1B ). The main atlantoaxial joint is formed by the inferior articular surfaces of C1 and the superior articular surfaces of C2 (see Fig. 9.1B ). A true synovial-lined joint also exists between the ventral dens and the dorsal surface of the C1 anterior arch and the dorsal aspect of the dens and the posterior ligamentous structures. The uncovertebral joints (joints of Luschka) exist only in the cervical spine below C2. The osseous uncinate processes arise from the dorsolateral margin of the superior end plates of the C3–C7 vertebral bodies and articulate with the level above: uncovertebral joints therefore exist from C2–C3 to C6–C7 (see Fig. 9.2 ). The joints of Luschka have features of both cartilaginous and synovial joints, and when degenerated, they can result in foraminal stenosis and even central stenosis. As their names imply, the costovertebral and costotransverse joints are articulations between the ribs (costo-) and the vertebral bodies or transverse processes of the thoracic spine ( Fig. 9.4 ).

The facet joints are the most prevalent joint in the spinal column and are formed by the inferior and superior articular processes of adjacent vertebral bodies. The facet surfaces (named relative to the joint space as described below) are covered with articular cartilage that allows for bending motion and offers some protection to shearing forces. The joints are encapsulated by a true synovial lining and loose capsular ligaments. In the cervical spine, there is a thick fibrous capsule laterally under which a small synovial recess may protrude. In the lumbar spine, a thick fibrous capsule is present along the posterior margin of the facet joint. The inferior synovial recess occurs at the caudal extent of this capsule and is the common location for access to the joint space. A complete discussion of the innervation of the facet joints is beyond the scope of this chapter. In general, the facet joints are dually innervated from paired medial branches of the dorsal primary rami. This dual innervation explains why complete denervation of a symptomatic facet joint requires treatment of both medial branches. Knowledge of the different facet joint orientations is important when planning facet joint interventions. The cervical facet joints are obliquely oriented from superior to posterior with a ventral to dorsal angle when viewed in the sagittal plane ( Fig. 9.5A ). The thoracic facet joints are oriented in the coronal plane limiting access for percutaneous procedures (see Fig. 9.5B ). The lumbar facet joints have a lunate configuration with the posterior margin oriented in the oblique sagittal plane and the anterior margin oriented in the oblique coronal plane (see Fig. 9.5C ). Access to the joint under fluoroscopy is accomplished from a shallow oblique sagittal projection.

Transverse Foramen, Intervertebral Foramen, and Nerve Roots
The transverse foramen, also known as the vertebral foramen or foramen transversarium, occurs in the cervical spine from C1 to C7. The transverse foramina develop when the neural processes posteriorly fuse with the vestigial costal element anteriorly. The contents of the transverse foramina include the vertebral artery, vertebral venous plexus, fibers of the sympathetic chain, and fat. Typically round or oval, these foramina vary in size and shape and often reflect the underlying size of the traversing vertebral artery. The vertebral artery typically enters the foramen at C6, but it can enter as high as C3. In the sagittal view, the vertebral artery is a few millimeters ventral to the adjacent exiting nerve root ( Fig. 9.6 ).

In the cervical spine, the intervertebral foramen runs obliquely anterolaterally. It is bounded by the pedicles, uncinate process, vertebral body, and superior articular facet. The exiting cervical nerves are positioned posteroinferiorly in the intervertebral foramina (see Fig. 9.6 ). Small foraminal veins connecting the epidural venous plexus and the anterior longitudinal intraspinal venous channels with the perivertebral venous plexus within the transverse foramina traverse the intervertebral foramen ( Fig. 9.7 ). There are eight paired cervical nerve roots, the first exiting the spinal canal between the skull base and C1. Therefore, in the cervical spine, the number of the nerve root passing through the foramen is one greater than the number of the pedicle that it passes beneath. For example, the nerve root passing through the intervertebral foramen at C3–C4 is the C4 nerve root.

The thoracic spine intervertebral foramina are rather constant, bounded by the pedicles, vertebral body, disc, and superior articular process of the vertebra below. The thoracic spinal nerves are more closely associated with the superiorly positioned articular process compared to the cervical spine. Small veins run through the intervertebral foramina as in the cervical spine. The exiting nerve roots are designated by the pedicle under which they immediately course. For example, at the T8–T9 level, the T8 spinal nerve root exits.
Much like the thoracic spine, the lumbar spine intervertebral foramina are bounded by the pedicles, vertebral body, disc, and superior articular process. The spinal nerve roots exit at a 45-degree angle inferolaterally and are closely associated with the medial and inferior margins of the pedicle under which they exit ( Fig. 9.8 ). The spinal nerve roots are numbered as in the thoracic spine; the numbered root exits below the same numbered pedicle. For example, at the L4–L5 level, the L4 spinal nerve exits.

Throughout the spine, the exiting nerve roots are composed of a smaller, ventral motor root and a larger, dorsal sensory root. The dorsal root contains a ganglion that can range in size from 5 to 15 mm. This dorsal root ganglion (DRG) lies within the intervertebral foramen and is most apparent in the lumbar and sacral spine. Small arterial branches from the lumbar arteries supply the DRG and have a fenestrated capillary endothelium. This anatomic configuration results in normal enhancement of the DRG on contrast examinations (see Figs. 9.8 and 9.9 ).

When contemplating a transforaminal or periganglionic intervention in the thoracolumbar region, one must consider the potential complication resulting from damage to the artery of the lumbar enlargement (artery of Adamkiewicz). This artery is the primary supply to the lower two-thirds of the spinal cord and enters the spinal canal via an intervertebral foramen. Although it typically enters on the left from T9–L1, the artery of Adamkiewicz can enter on either side from T5–L4. The artery usually runs in the more superior and ventral aspect of the foramen ( Fig. 9.10 ). Techniques to improve the safety of epidural steroid injections have been recently described.

Intervertebral Discs
Intervertebral discs separate the vertebral bodies and contribute a significant proportion (20%–35%) of the height to the spinal column. The discs are thicker in the cervical and lumbar regions and thicker anteriorly than posteriorly, contributing to the lordotic curvatures of the spine in these regions. The primary function of the disc is to absorb the impact of daily axial loading and confer some flexibility. Discs are composed of three main components: the nucleus pulposus, annulus fibrosis, and the cartilaginous end plate. The nucleus pulposus contains type II collagen, hyaluronic acid, and glycosaminoglycans. This composition confers excellent compressive resistance and, when hydrated, has characteristic imaging findings on magnetic resonance imaging (MRI). The annulus fibrosis consists of an outer dense circumferential fibrous band and an inner fibrocartilaginous layer. The outer layer fibers, also known as Sharpey fibers, insert into the ring apophyses. The cartilaginous end plate is composed of hyaline cartilage that tightly adheres to the vertebral end plate. Vascular supply to the disc is primarily via small nutrient channels through this cartilaginous end plate.
Ligaments
Ligaments of the spine provide stability while allowing flexion, extension, and rotation. There are five main ligamentous structures throughout the spinal column: anterior longitudinal ligament (ALL), posterior longitudinal ligament (PLL), ligamentum flavum, interspinous ligaments, and supraspinous ligament. The ALL and PLL run along the anterior and posterior margins of the vertebral bodies, respectively ( Fig. 9.11 ). The ALL adheres to the vertebral body and intervertebral discs. The PLL adheres to the annulus fibrosis of the disc but does not contact the posterior vertebral margin to any significant degree. The ligamentum flavum runs along the length of the spinal canal extending between adjacent laminar segments and defining the dorsolateral margins of the spinal canal. The interspinous ligaments run between adjacent spinous processes, whereas the supraspinous ligament runs along the tips of the spinous processes.

Specialized ligaments are present at the craniocervical junction, including the atlanto-occipital ligament, apical ligament, tectorial membrane, and the cruciate ligaments that form the transverse ligament. These ligaments provide stability and flexibility at the craniocervical junction. Further discussion of these ligaments is beyond the scope of this chapter.
Imaging Overview
Conventional Radiographs (X-Rays)
Conventional or plain radiographs record differential attenuation of the x-ray beam by tissues based on their differential densities. For example, the cortical bone is very dense and almost completely attenuates the beam. The heart is soft tissue and partially attenuates the beam, and the lung is mostly air, thus attenuating very little of the beam. Conventional radiographs are quick, inexpensive, and easy to perform and have excellent spatial resolution. Important information about the spine can be obtained with con ventional radiographs, including alignment, structure, and mineralization. Dynamic, weight-bearing upright flexion and extension views can reveal a stable or unstable spine in chronic and acute scenarios. This is the most commonly available modality that allows for this type of stress-related imaging. Osseous foraminal stenosis and spondylolysis can be diagnosed with oblique projections. Vertebral fractures and joint dislocations can be detected, although acuity can be difficult to discern and details are not as well characterized as with computed tomography (CT). Although conventional radiographs are far less optimal than CT for soft tissue evaluation, degenerative changes of the disc can be identified such as disc dehydration (air in disc) and disc collapse.
Standard frontal anterior-posterior (AP) (including odontoid view when imaging the cervical spine) and lateral projections are the minimum required for adequate evaluation ( Figs. 9.12A–C, 9.13A, B, 9.14A–C ). In the cervical and lumbar regions, oblique projections are helpful in evaluating the facet joints, articular processes, and intervertebral foramina (see Figs. 9.12D, E, 9.14D, E ). When spondylolisthesis or spondylolysis is present, or with fractures and/or ligamentous disruption, flexion and extension views aid in demonstration of abnormal motion. Flexion and extension views may be supplemented by direct real-time observation using fluoroscopy.



Plain films can detect changes related to systemic diseases such as ankylosing spondylitis and diffuse sclerotic/lytic states ( Fig. 9.15 ). Also, there is no good substitute for plain radiographs to evaluate overall alignment abnormalities in patients with extensive kyphoscoliotic deformities.

Conventional radiography is the easiest and most cost-effective method of assessing alignment and structure of the spine in both traumatic and nontraumatic conditions. On lateral projection, three longitudinal curves may be used to evaluate alignment of the vertebrae ( Fig. 9.16 ). The anterior and posterior spinal lines trace the course of the anterior and posterior longitudinal ligaments, respectively. The spinolaminar line traces the course of the ligamentum flavum along the deep surface of the laminae. On frontal projection, a vertical line drawn through the tips of the spinous processes serves as a reference for evaluation of lateral curvature ( Fig. 9.17 ). The relationship of this line and the pedicles demonstrates rotational malalignment.


Plain radiographs can easily depict the failure of implanted hardware, such as screw loosening and rod fractures. Even known hardware fractures can be difficult to detect with CT due to beam-hardening artifacts that can obscure large portions of the images.
Myelography and Postmyelography Computed Tomography
Myelography is the radiographic technique used to evaluate the contents of the spinal canal by the introduction of a nonionic, water-soluble, radiographically dense iodinated contrast material into the spinal subarachnoid space. This contrast material outlines the spinal cord and nerve roots, which appear as filling defects in the radio-dense contrast column on conventional radiographs. Extradural indentations into the contrast column are observed and generally represent disc abnormalities, ligament thickening, or hypertrophic facet degenerative changes. Spinal stenosis can be diagnosed and nerve root impingement can be detected. Redundant thickened nerve roots and arachnoiditis can also be demonstrated ( Fig. 9.18 ). Myelography should always be followed by a postmyelography CT scan to provide better definition of anatomic relationships of the contents of the spinal canal to the surrounding structures.

The use of myelography has decreased significantly due to the relatively invasive nature of the procedure and the availability of other noninvasive imaging tools, including CT and MRI, which provide excellent spatial and contrast resolution. The risks of myelography are directly related to the lumbar puncture (LP) and injection including positional headache, contrast-related seizure, and infection. The most common of these complications is the post-LP positional headache. If this headache does not respond to conservative therapy, an epidural blood patch can be performed for more definitive treatment. Seizures related to intrathecal contrast administration are uncommon, but the seizure threshold decreases with certain medications including numerous antidepressants. In general, patients should be screened for specific medications and rescheduled if they are found to be on any seizure threshold–reducing medications. Myelography is now used mainly as a problem-solving tool when CT or MRI examination cannot be performed due to contraindications, are equivocal, or are limited due to artifacts from surgical hardware.
Computed Tomography
CT is an x-ray technique that is considerably more sensitive to the differential attenuation of the x-ray beam than plain film radiography. CT provides the best possible definition of osseous structures and has excellent spatial resolution. The newest generation of CT scanners employs slip-ring technology (helical acquisition), multidetector systems, high-speed rotation, and dynamic table translation to optimally image the spine. Dose-reduction software now changes the patient dose “on the fly”: The current (mA), and therefore the dose, changes in response to the thickness of the individual patient at each slice. Overlapping data sets can be acquired that allow for multiplanar reformatting, and three-dimensional data sets can be acquired for volumetric analysis or volume rendering applications.
As with conventional radiographs, CT imaging is based on differential attenuation of the x-ray beam but can differentiate not only bone from soft tissue but also between different densities of bone and soft tissue structures. Differences in radiographic density of ligament, disc material, and cerebrospinal fluid (CSF) make identification of disc herniations and ligamentous disorders possible using CT ( Fig. 9.19 ). Subtle areas of bone sclerosis or lysis can easily be displayed with CT. Windowing techniques used in the display of CT images allow optimal viewing of image data depending on the tissue type of interest. The administration of intravenous iodinated contrast material may be valuable in certain circumstances to highlight vascular structures, such as the epidural venous plexus or adjacent arteries, or to demonstrate abnormal soft tissue enhancement (tumor, inflammation, infection).

Artifacts from metallic surgical implants, such as spinal rods, transpedicular screws, laminar wires/hooks, and intervertebral/vertebral body cages, can severely limit the diagnostic value of CT images. In these cases, conventional radiographs and myelography may prove to be the best diagnostic imaging modalities. Even this limitation improves as CT scanners evolve and postprocessing techniques improve, such as CT metal artifact reduction with iterative reconstruction technique. Compared to several years earlier, there are now 256-slice and 320-slice multidetector CT scanners in existence that provide increasingly higher spatial resolution. There are also dual source CT scanners with greater capability for differentiating attenuation among different soft tissue, osseous, and metallic structures. The radiation dose from CT can be several times that of plain radiography, depending on technique and protocols. Hence, appropriate care should be exercised in using it in the more sensitive populations, including children, pregnant females, and other young adults.
Magnetic Resonance Imaging
MRI uses strong magnets, gradient fields, and radiofrequency waves to localize and characterize tissues based on the amount and state of the ubiquitously present hydrogen atoms (protons). There is no ionizing radiation employed with MRI, but there are risks including those related to energy deposition, electrical and metal implants, and an unknown/unquantified risk to the fetus. The very good soft tissue contrast resolution afforded by MRI and its multiplanar tomographic capability make it the most versatile and useful diagnostic imaging modality for spinal disorders. It provides a wide field of view with excellent definition of tissue types, such as bone marrow, muscle, ligament, disc material, and nerve roots. MRI allows precise definition of extradural, intradural extramedullary, and intramedullary pathology. Evaluation of medullary bone with MRI is excellent, and many osseous conditions resulting in marrow edema or marrow replacement (e.g., metastatic disease and myeloma) are well demonstrated. However, demonstration of dense cortical bone, sclerotic lesions, and osteophytes is less precise than by CT.
Standard MRI protocols usually include sagittal and axial images with T1- and T2-weighted sequences. T1 weighting provides excellent anatomical delineation. In general, high signal intensity on T1 represents fat (such as in fatty bone marrow, subcutaneous fat), whereas low signal intensity represents fluid (such as CSF, bone marrow edema, and normal nucleus pulposus) ( Fig. 9.20A ). T2 weighting makes fat-containing structures less bright than on T1 and makes fluid-containing structures hyperintense (bright) (see Fig. 9.20B ). Soft tissue structures such as muscles and spinal cord have intermediate signal intensities on T1 and T2 sequences. The short-tau inversion recovery (STIR) sequence is a fat-suppressed, T2-weighted sequence that is extremely sensitive to minute amounts of fluid (see Fig. 9.20C ). This sequence is particularly useful in detecting edema as can be seen with traumatic injury, malignancy, and infection. Gradient recalled echo (GRE) T2-weighted imaging is sensitive to blood products and calcium and is particularly useful in the setting of spine trauma for evaluating the spinal cord (see Fig. 9.20D ). When evaluating scoliosis, coronal T1- or T2-weighted imaging may be added to better assess the extent of curvature (see Fig. 9.20E ).

In the cervical spine, thin-section axial two- or three-dimensional GRE T2 images are used to further evaluate central canal and intervertebral foraminal stenosis. The degree of stenosis produced by osteophytes may be exaggerated on the GRE T2 sequence because of the sensitivity to susceptibility artifacts. Proton density (intermediate T2) and T2-weighted axial images are used in the lumbar region. Whether using a GRE T2 axial image in the cervical spine or a spin echo T2-weighted axial image in the thoracic or lumbar spine, the effect is the same: a “myelographic” effect is produced with hyperintense CSF within the thecal sac surrounding the intermediate signal intensity of the spinal cord and nerve roots ( Fig. 9.21 ).
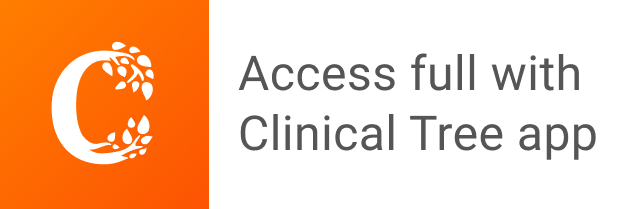