Abstract
For the survival and well-being of the organism, the detection of tissue-damaging or potentially tissue-damaging threats in the environment is essential. This critical function is served by the nociceptive system, which consists of peripheral, small myelinated, and unmyelinated nociceptive afferent neurons that project to the spinal cord dorsal horn where second-order neurons process the incoming nociceptive information. Axons from spinal projection neurons travel through the anterolateral tract of the spinal cord and project to supraspinal sites in the brainstem, midbrain, and diencephalon, where they terminate in the hypothalamus and different regions of the thalamus. Third-order neurons send axons to cortical areas, including the cingulate and the insula, and subcortical structures, such as the limbic system. Nociceptive signaling includes transduction of noxious stimuli to action potentials in the periphery, transmission of these nerve signals to the central nervous system, modulation of the sensory information at multiple sites along the nociceptive pathway, and finally the perception at the level of the brain. Nociceptive information is modulated in the spinal cord by descending inhibitory as well as facilitatory input from supraspinal regions, such as the rostroventral medulla. Following tissue injury, the sensitivity of peripheral and central neurons may increase (“peripheral” and “central” sensitization) and descending inhibitory or facilitatory modulation may change resulting in an increase in pain sensation (“hyperalgesia”). Preclinical studies suggest that neuroimmune interactions involving nonneuronal glial cells, astrocytes, and microglia may also play a role in pain modulation. While these plastic changes serve to increase the protection of the organism, persistent maladaptive changes may result in chronic pain.
Keywords
dorsal horn, hyperalgesia, modulation, nociceptor, sensitization, supraspinal
Pain is a physiologic consequence of tissue injury and serves a vital protective function. For example, clinical observations of patients with congenital insensitivity to pain and patients with leprosy have clearly demonstrated that the absence of pain results in repeated injuries and disabilities. However, pain can become a disease when it occurs or persists in the absence of tissue damage or following appropriate healing of injured tissues. This chronic pain is disabling, has considerable negative impact on quality of life of the individual, and has profound economic impact on the family and society.
The International Association for the Study of Pain defines pain as “an unpleasant sensory and emotional experience associated with actual or potential tissue damage, or described in terms of such damage.” This definition acknowledges that pain is not only a sensory experience but may be associated with affective and cognitive responses. The definition also recognizes that the intensity of pain and the severity of tissue damage are not necessarily correlated. Thus, an understanding of the anatomic substrates and physiologic mechanisms by which noxious and nonnoxious stimuli are perceived provides the essential background to apprehend the mechanisms of acute and chronic pain and the sites of action of pharmacologic therapies for pain.
Somatosensation, Nociception, and Pain
Somatosensation is the physiologic process by which neural substrates are activated by physical stimuli resulting in the perception of what we describe as touch, pressure, and pain. Nociception is the physiologic process of activation of neural pathways by stimuli that are potentially or currently damaging to tissue. A stimulus is considered nociceptive when it induces behavioral avoidance or escape response in animals and humans or when the stimulus evokes activity in specialized groups of afferent fibers (i.e., nociceptors). Clinically, the degree of nociception is inferred by overt evidence of tissue damage. In contrast to nociception, pain is a conscious experience. Although the stimulus-induced activation of afferent neural pathways plays an important role, other factors such as alterations in somatosensory processing after injury to tissues and/or nerves and psychosocial factors may influence the overall perception of pain. The experience of pain, particularly chronic pain, often results in suffering. Suffering results from a multitude of factors that includes loss of physical function, social isolation, family distress, and a sense of inadequacy or spiritual loss. This chapter briefly reviews the basic anatomy and physiology of the neural pathways that respond to somatosensory stimuli, especially nociceptive stimuli, and emphasizes the plasticity in this system following an injury. This knowledge is fundamental in the evaluation and subsequent management of patients with painful disorders.
The sequence of events by which a stimulus is perceived involves four processes: (1) transduction, (2) transmission, (3) modulation, and (4) perception ( Fig. 1.1 ). Transduction occurs in the peripheral terminals of primary afferent neurons, where different modes of stimuli (e.g., mechanical, heat, chemical, or cold) induce a generator potential through activation of transduction channels expressed on the axonal membrane. If the generator potential is sufficient, it will generate action potentials that are then transmitted through the nervous system. There are three major components of the transmission system. The peripheral sensory neurons with their cell bodies residing in the dorsal root ganglia transmit impulses from the site of transduction at their peripheral terminal to the spinal cord where the central terminals synapse with second-order neurons. The spinal neurons are the second component in the transmission network. These cells send projections to the thalamus and various brainstem and diencephalic structures. Finally, neurons of the brainstem and diencephalon form the third component of the transmission network as they project to various cortical sites. Modulation is the process whereby neural activity may be altered along the pain transmission pathway. A major site of modulation occurs within the dorsal horn of the spinal cord. Modulation at this level of processing involves a multitude of neurotransmitter systems that will be discussed in Chapter 2 . Activation of pain modulation systems usually results in less activity in the pain transmission pathway after a noxious stimulus. Examples of activation of this process include stress-induced analgesia. However, in some circumstances, modulation can also result in an enhancement of pain signaling. Perception is the final stage of the nociceptive process by which neural activity in the somatosensory transmission pathway results in a subjective sensation of pain. It is presumed that this process results from the concerted activation of primary and secondary somatosensory and limbic cortices.

Peripheral Mechanisms
In general, somatosensation begins with activation of primary afferent neurons. These neurons are part of the peripheral nervous system, with cell bodies located in the dorsal root ganglia, from which the neurons project with a peripheral axon into the target tissue (skin, muscles, joints) and a central axon into the spinal cord. Primary afferent fibers are classified based on their conduction velocity and the stimuli by which they are activated. Much of our knowledge about the physiology of primary afferents including nociceptors originates from studies on cutaneous afferents (i.e., those that innervate the skin). Many of the principal findings made on cutaneous afferents extend to primary afferents innervating other peripheral tissues. Among cutaneous afferents, three classes of primary afferent fibers can be differentiated. The fastest-conducting fibers (>20 m/s) are the large-diameter myelinated A beta (Aβ) fibers. These afferents are activated by nonnociceptive stimuli that evoke the sensation of light touch, pressure, or hair movement. The axons of nociceptive neurons are generally thinly myelinated A delta (Aδ) fibers or unmyelinated C fibers, which conduct between 2 and 20 m/s and less than 2 m/s, respectively. Many nociceptors have the capacity to respond to intense heat, cold, mechanical, and chemical stimuli (i.e., they respond to different stimulus modalities and are therefore “polymodal”). The functional role of the Aδ- and C-fiber nociceptors may be different. The C fibers are the predominant (75%) type of afferent fiber in peripheral nerves. Recordings from C fibers in humans suggest that C-fiber activity is associated with a prolonged burning sensation. In contrast, activation of faster-conducting Aδ fibers evokes a sharp, intense, tingling sensation. The combined activation of these two groups of afferents, such as by an intense brief heat stimulus, results in a dual-pain sensation as Aδ fibers convey the rapid-onset first pain sensation, a pricking pain, whereas C fibers mediate the slower-onset, burning second pain sensation that follows brief intense heat stimulation to the skin. Combined, Aδ- and C-fiber nociceptors encode and transmit information to the central nervous system (CNS) concerning the intensity, location, and duration of noxious stimuli. Although polymodal nociceptors are the most common type, a functionally distinct and important subgroup of nociceptors is insensitive to mechanical stimuli. These mechanically insensitive nociceptors (“MIAs”) likely act as chemonociceptors, and input from these afferents is thought to be crucial for the induction and maintenance of central sensitization.
Nociceptive afferents are further subclassified based on the molecules expressed on their cell surface (e.g., receptors, glycoconjugates), based on the molecules they store and release (e.g., peptides), and based on the enzymes they contain. Although none of these cell markers is completely specific for the peripheral target tissue innervated, the percentage of dorsal root ganglion cells positive for a given marker differs significantly among target tissues. For example, almost all visceral afferents are peptidergic, but only approximately half of the afferents projecting to the skin are, and only a small percentage of the nonpeptidergic afferents, characterized by binding the plant isolectin B4 (IB4) from Griffonia simplicifolia, project to muscle. Similarly, the central projection areas of peptidergic and nonpeptidergic afferents differ, with peptidergic fibers mainly projecting to lamina I and lamina II outer, and IB4 binding (nonpeptidergic) afferents projecting preferably to lamina II inner (e.g., Silverman and Kruger, but see also Woodbury et al. ). Most peptidergic neurons express the tyrosine kinase receptor A (trk A), suggesting that they depend on nerve growth factor (NGF) for survival. In contrast, most IB4-positive dorsal root ganglion cells do not express trk A but express one of the glial cell-derived neurotrophic factor (GDNF) family receptors (GDNFRa1–4) together with receptor tyrosine kinase Ret. Peptidergic and nonpeptidergic neurons also express different patterns of receptors involved in signal transduction, and they may therefore display different sensitivities to a given stimulus. Thus the P2X3 receptor, which mediates nociceptor excitation by ATP, is primarily expressed in IB4-positive neurons. In contrast, the vanilloid receptor 1 (VR1/TRPV1), which mediates responses to heat, capsaicin, and protons, is expressed in only a minority of IB4-positive cells in mice, and IB4-positive neurons are less responsive to these stimuli than their IB4-negative counterparts. The role of these various peptides and receptors, in addition to others, in pain transmission is discussed in greater detail in Chapter 2 . Another class of receptors primarily expressed in small primary afferent neurons are mas-related G protein–coupled receptors (Mrgprs). In mice, different Mrgprs are expressed in nonoverlapping neuronal populations, some of which have been linked to mediating specific behavior. For example, MrgprD neurons are thought to mediate nociceptive behavior to mechanical stimuli, whereas neural activity in neurons expressing MrgprA3 induces scratching behavior in response to itch-producing stimuli. In primary afferent nerve fibers, including nociceptors, information on the intensity of a given stimulus is coded by the number of action potentials in a population of primary afferents, with a generally monotonic relationship between the stimulus intensity and the number of impulses generated by afferent fibers. The sensitivity of nociceptors to different stimuli is not fixed but can change considerably under pathologic conditions. For example, mediators released during inflammation can dramatically decrease the threshold for activation and increase responses to suprathreshold stimuli. This peripheral sensitization of nociceptive afferents is a major contributor to increased pain that can be elicited from a site of injury. In addition to this primary hyperalgesia from the injury site, increased pain responses may also be elicited from the surrounding area. This so-called secondary hyperalgesia involves mechanisms residing in the CNS and leads to the sensitization of central neurons (i.e., central sensitization).
Voltage-gated sodium channels are crucial for the generation and conduction of action potentials in central and peripheral neurons. The different channel isoforms (Na V 1.1-1.9) are broadly classified as tetrodotoxin (TTX) sensitive and TTX insensitive. Among these channels, the TTX-sensitive Na V 1.7 isoform and the TTX-insensitive isoforms (Na V 1.8, Na V 1.9) are of major interest in nociception and pain. Thus loss-of-function mutations in the gene (SCN9A) that encodes Na V 1.7 lead to congenital insensitivity to pain (CIP) and anosmia. Conversely, gain-of-function mutations in the same gene can lead to severe spontaneous pain episodes in patients, for example, with inherited erythromelalgia (IEM) or extreme paroxysmal pain disorder (for review see Dib-Hajj et al. ). Gain-of-function mutations of Na V 1.7 have also been observed in patients with small fiber neuropathy. In contrast to Na V 1.7, which, in addition to primary afferent fibers, is also expressed in sympathetic nerve fibers and olfactory epithelium, the TTX-insensitive isoforms Na V 1.8 and Na V 1.9 appear to be selectively expressed in nociceptive afferents. Similar to Na V 1.7, gain-of-function mutations in Na V 1.8 have been found to contribute to painful peripheral neuropathy, and a gain-of-function mutation for Na V 1.9 leads, paradoxically, to CIP. It should be noted that Na V 1.7, Na V 1.8, and Na V 1.9 differ significantly in channel properties, and they contribute differently to cell excitability and action potential generation (for review see Dib-Hajj et al. ).
Spinal Mechanisms
The first synapse in somatosensory processing of information from the body surface occurs at either the spinal dorsal horn or in the dorsal column nuclei at the spinal cord–brainstem junction. Somatosensory processing for information from the face is similarly processed either in the spinal trigeminal nucleus (pain and temperature) or in the chief sensory nucleus of the trigeminal nerve located in the mid-pons region of the brainstem. Both nociceptive and nonnociceptive fibers provide inputs to both of these initial targets. However, under normal circumstances the dorsal column nuclei and the chief sensory nucleus can be considered to selectively process inputs from large myelinated Aβ fibers related to light touch, whereas the spinal dorsal horn and spinal trigeminal nucleus process inputs of nociceptive Aδ and C fibers. This separation of modalities in the somatosensory system is the basis for the localization of neural lesions through neurologic examination in patients.
Nociceptive primary afferent fibers terminate in a highly ordered way in the spinal dorsal horn on the same side of the body of their origin. The dorsal horn is anatomically organized in the form of layers or laminae, as first recognized by Rexed in the cat ( Fig. 1.2 ). The unmyelinated C fibers terminate primarily in the most superficial lamina (I and II outer), whereas the thinly myelinated Aδ fibers end in lamina I, and in laminae III to V. Collaterals of the large myelinated fibers (Aβ) terminate in laminae III to V of the dorsal horn. Lamina I is also known as the marginal nucleus and lamina II as the substantia gelatinosa of Rolando.

Two predominant types of second-order nociceptive spinal and spinal trigeminal projection neurons have been identified: wide dynamic range (WDR) neurons and nociceptive-specific (NS) neurons. WDR cells are especially concentrated in the deeper laminae of the dorsal horn (III to V), where they receive input from both low-threshold Aβ and nociceptive Aδ and C fibers, and hence are activated by both innocuous and noxious stimuli. However, the responses of WDR cells to these stimuli are graded such that the noxious stimuli evoke a greater response than nonnoxious stimuli. WDR spinal projection neurons (i.e., neurons whose axons terminate in supraspinal targets) have a spontaneous discharge (average rate of approximately 11 Hz in monkeys), and their activity is increased by innocuous cutaneous (average rate of approximately 25 Hz after brushing skin with a hair brush), and noxious mechanical stimulation (approximately 50 Hz after a small arterial clip is applied to the skin) ( Fig. 1.3 ).

In contrast to WDR cells, NS projection cells respond only to noxious stimuli under physiologic conditions. The majority of NS cells are found in the superficial laminae of the dorsal horn (I and outer II). These cells have a lower rate of spontaneous activity than do WDR cells, averaging approximately 3 to 5 Hz. The discharge rates to the noxious stimuli of NS cells are comparable to those of WDR cells, averaging approximately 50 Hz ( Fig. 1.4 ).

The axons of both the WDR and NS second-order neurons cross the midline near the level of the cell body, gather into bundles of ascending fibers in the contralateral, anterolateral spinal region, and then ascend toward targets in the brainstem and diencephalon ( Fig. 1.5 ). The conduction velocity of the WDR cells is usually faster than that of the NS cells (approximately 30 m/s vs. 12 m/s). In addition, the axons of the NS cells that largely arise from laminae I of the dorsal horn and those of the WDR cells arising primarily from laminae III to V tend to run in slightly different positions in the anterolateral spinal funiculus. In the anterolateral spinal column, the NS cell axons are found in the dorsal medial region, whereas axons of WDR cells are more concentrated in the ventral lateral region.

Spinal Modulation
The concept of modulation of noxious inputs at spinal levels was highlighted by the gate control theory of Melzack and Wall. This theory suggested that input along low-threshold (Aβ) fibers inhibits the responses of WDR cells to nociceptive input. The theory was offered as an explanation for the efficacy of transcutaneous electrical stimulation for pain relief. Subsequent studies have identified intrinsic spinal neurons that release a plethora of neurotransmitters in the spinal cord that play a role in the modulation of nociceptive impulses. Furthermore, a number of inputs to the dorsal horn from various brainstem sites have been shown to also modulate peripheral inputs, as well as outputs, of intrinsic cells. Both types of modulation, that arising in the local network of cells at the spinal levels as well as that from the descending inputs, can result in either augmented or inhibited output from spinal cord nociceptive neurons. It is the combined effects of spinal excitatory and inhibitory systems that determine what messages are delivered to the higher levels of the CNS.
A special type of spinal modulation that is observed under certain circumstances is known as central sensitization. In this phenomenon, the capacity for transmission in the nociceptive system is changed or shows neuronal plasticity. The result of this plasticity is that after a noxious stimulus of sufficient intensity and duration, such as a surgical incision, the coding of pain-signaling neurons for a given stimulus may be increased. One example of central plasticity is the phenomenon of wind-up, whereby repeated stimulation of C fibers at intervals of 0.5 to 1 Hz results in a progressive increase in the number of discharges evoked by each volley. In addition to an increase in discharges evoked by a given stimulus, sensitized spinal neurons also show an expansion of receptive field size and an increase in spontaneous discharge rate. WDR cells tend to become sensitized more readily than NS cells. However, in circumstances in which NS cells do show sensitization, they often acquire novel responsiveness to innocuous stimuli and hence could be recategorized as WDR neurons. The neurochemistry of central sensitization is discussed in Chapter 2 . Better understanding of the pharmacology of this and other types of plasticity will have profound consequences in the development of new analgesic pharmacotherapies.
A different form of spinal modulation is exerted by glial cells (microglia, astrocytes, and oligodendrocytes). Microglia, the macrophages of the CNS, and astrocytes become activated after different insults including nerve injury, inflammation, or chronic opioid therapy. Upon activation, these cells can release a number of substances, including cytokines, inflammatory mediators, and growth factors, which then can affect neuronal function in multiple ways (for review see Ji et al. and Tiwari et al. ). After nerve injury, for example, activated microglia release brain-derived neurotrophic factor (BDNF), which leads to the downregulation of potassium-chloride cotransporter KCC2 in lamina I neurons. As a consequence, a shift in transmembrane anion gradient can occur such that normally inhibitory input becomes excitatory. Although animal studies have provided substantial evidence for a role of glia in chronic pain, it should be noted that the contribution and role of glia in chronic pain states in humans are less clear.
Supraspinal Mechanisms
Supraspinal structures involved in somatosensory processing include brainstem, diencephalic, and cortical sites. There are two sets of somatosensory inputs to the brainstem and diencephalon. First, many axons and axon collaterals of the spinal projection neurons that ascend in the anterolateral spinal quadrant depart this ascending tract to terminate in a number of nuclei of the brainstem and midbrain (see Figs. 1.5 and 1.6 ). These target sites include brainstem autonomic regulatory sites that influence cardiovascular and respiratory functions, whereas in the midbrain there are multiple inputs to centers from which both descending and ascending (e.g., to thalamus) modulation of somatosensory processing is evoked. The remainder of the so-called anterolateral system fibers continue through the brainstem and midbrain to terminate in diencephalic structures, including the hypothalamus and posterior, lateral, and medial regions of the thalamus (see Figs. 1.5 and 1.6 ).

The second set of somatosensory inputs to the brainstem includes those primary afferent fibers that ascend in the dorsal (posterior) columns of the spinal cord to form their first synapse at the dorsal column nuclei. These inputs are organized so that the fibers from the lower extremities synapse most medially in the nucleus gracilis and inputs from the upper extremities synapse laterally in the nucleus cuneatus. The trunk is represented in regions of both nuclei. Comparative inputs from the face are processed in the chief sensory nucleus of the trigeminal nerve located at the origin site of cranial nerve five in the mid-pons of the brainstem. The axons of the second-order cells in the dorsal column nuclei cross the midline and form the medial lemniscus on the contralateral side of the brainstem. These fibers then ascend through the brainstem and midbrain, acquiring the functionally related fibers from the trigeminal nerve as they pass and continue on to provide the second somatosensory input to the diencephalon as they terminate in the ventral posterior lateral (VPL) nucleus (inputs from the body) and ventral posterior medial (VPM) nucleus (inputs from the face) of the thalamus.
The somatosensory inputs to the cortex include the third-order projections from thalamic somatosensory relay neurons of VPL and VPM as well as third- (and higher-) order neurons projecting from brainstem and midbrain relay neurons. Some of these projections are highly organized and quite specific. For example, the cells in the core of VPL that receive inputs from the dorsal column–medial lemniscus fibers project to cortical areas SI and SII. The neurons in the posterior region of the lateral thalamus that receive inputs from the anterolateral system project to SII and the retroinsular areas of cortex, whereas medial thalamic nuclei ultimately project to the anterior cingulate cortex. Similarly, somatosensory relay neurons of the midbrain parabrachial nucleus project specifically to the amygdaloid nucleus of the neocortex. On the other hand, other third-order projections into cortex are quite diffuse. Outputs from cells of the brainstem reticular activating system that receive somatosensory inputs from the spinoreticular tract, for example, project throughout the neocortex.
In addition to peripheral and spinal mechanisms of nociceptive processing and modulation, there are several cortical regions that consistently have been shown to be involved in acute and chronic pain states. Although the exact brain areas included in what has been coined the “pain matrix” have been the focus of debate, the primary and secondary somatosensory cortices, insula, anterior cingulate cortex, prefrontal cortex, and several nuclei of the thalamus have consistently been shown to be active in imaging studies of acute and chronic pain states (see Fig. 1.6 ). In addition, pharmacologically induced analgesia has been shown to have effects in these brain regions. The “pain matrix” has further been categorized as comprising the lateral pathway, which encodes for the sensory-discriminative aspect of pain perception, and the medial pathway, which encodes for the affective component of pain perception. Brain structures involved in the affective component of pain processing are required for encoding the unpleasant and aversive aspects of pain, which is critical for self-preservation. A case study of several patients with unilateral ischemic damage to the insular cortex exhibited pain asymbolia, as evidenced by a lack of or inappropriate emotional response to multiple painful stimuli applied over the entire body. Moreover, these patients failed to learn appropriate escape or protective responses in response to the painful stimuli. Another example of the role of cortical structures in the experience of pain is the placebo analgesic effect. Previous studies have shown that the placebo effect is at least partially mediated by activation of the endogenous opioid system, and μ-opioid receptors are highly localized within structures of the pain matrix. Studies using positron emission tomography and the selective μ-opioid radiotracer [ 11 C] carfentanil have shown that the placebo-mediated activation of the endogenous opioid system is predominantly located in the pain matrix structures, such as the anterior cingulate, prefrontal cortex, insula, medial thalamus, amygdala, and periaqueductal gray (PAG).
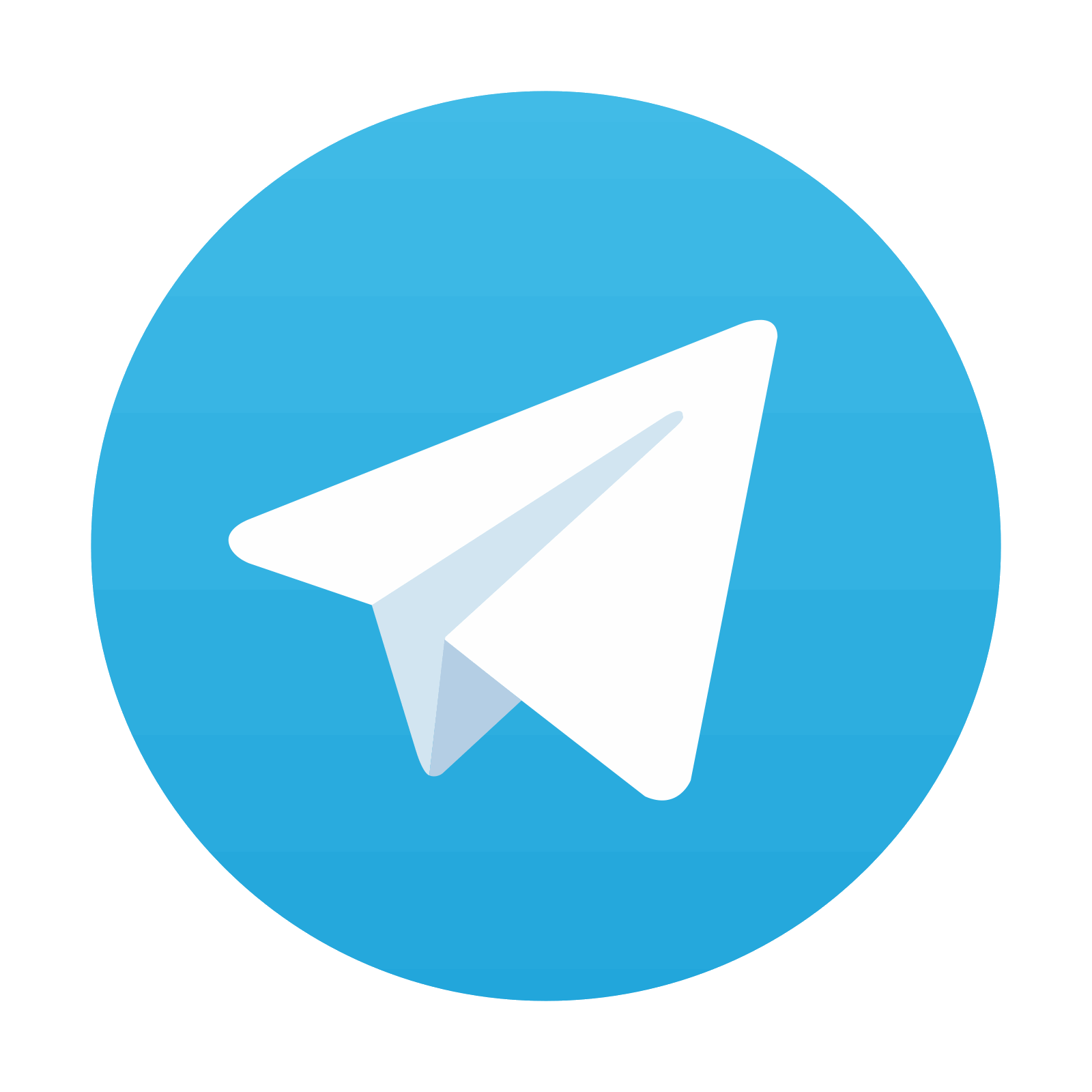
Stay updated, free articles. Join our Telegram channel
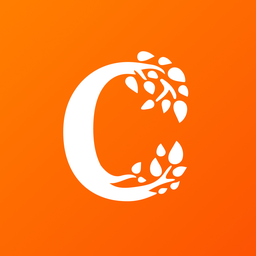
Full access? Get Clinical Tree
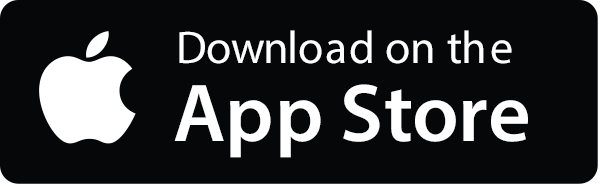
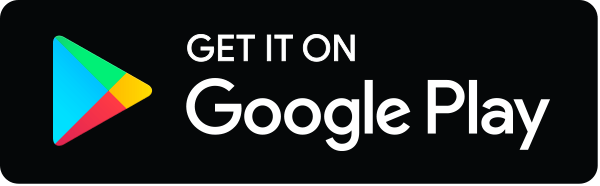