Chapter 74 A Brief Introduction to Oceanography
Water Characteristics
Heat capacity is a measure of the heat required to raise the temperature of 1 gram of a substance by 1° C. Different substances have different heat capacities, and not all substances respond to identical inputs of heat by rising in temperature the same number of degrees (Table 74-1). Heat capacity is measured in calories per gram per degree centigrade.
TABLE 74-1 Heat Capacity of Common Substances*
Substance | Heat Capacity† in Calories/Gram/° C |
---|---|
Silver | 0.06 |
Granite/sand | 0.20 |
Aluminum | 0.22 |
Alcohol (ethyl) | 0.30 |
Gasoline | 0.50 |
Acetone | 0.51 |
Ice (not freezing or thawing) | 0.51 |
Pure liquid water | 1.00 |
Ammonia (liquid) | 1.13 |
* Heat capacity is a measure of the heat required to raise the temperature of 1 gram of a substance by 1° C.
† Different substances have different heat capacities. Note how little heat is required to raise the temperature of 1 gram of silver by 1° C. Of all common substances, only liquid ammonia has a higher heat capacity than liquid water.
Because about 3.5% of seawater consists of dissolved substances, boiling away 100 kg of seawater theoretically produces a residue with a mass of 3.5 kg. Because variations of 0.1% are significant, however, oceanographers prefer to use the parts-per-thousand notation (‰) rather than percent (%, parts-per-hundred notation) when discussing these materials. The seven ions listed below oxygen and hydrogen in Table 74-2 make up more than 99% of this residual material; sodium and chloride make up 85% of the total. When seawater evaporates, its ionic components combine in many different ways to form table salt, Epsom salts, and other mineral salts.
TABLE 74-2 Major Constituents of Seawater at 34.4‰ Salinity
Constituent | Concentration in Parts per Thousand (‰) or Grams per Kilogram (g/kg) | Percent by Mass |
---|---|---|
Water Itself | ||
Oxygen | 857.8 | 85.8 |
Hydrogen | 107.2 | 10.7 |
Most Abundant Ions | ||
Chloride (Cl−) | 18.980 | 1.9 |
Sodium (Na+) | 10.556 | 1.1 |
Sulfate (SO42-) | 2.649 | 0.3 |
Magnesium (Mg2+) | 1.272 | 0.1 |
Calcium (Ca2+) | 0.400 | 0.04 |
Potassium (K+) | 0.380 | 0.04 |
Bicarbonate (HCO3−) | 0.140 | 0.01 |
Total | 999.377 g/kg | 99.99% |
Ocean Structure
Heat combines with salinity to define ocean structure. A liter of seawater weighs between 2% and 3% more than a liter of pure water because of the solids (often called salts) dissolved in seawater. The density of seawater is thus between 1.020 and 1.030 g/cm3 compared with 1.000 g/cm3 for pure water at the same temperature. Cold, salty water is denser than warm, less salty water. Seawater’s density increases with increasing salinity, increasing pressure, and decreasing temperature. Figure 74-1 shows the relationship between temperature, salinity, and density. Notice that two samples of water can have the same density at different combinations of temperature and salinity.
Figure 74-2 contrasts polar, tropical, and temperate thermal profiles, showing that the thermocline is primarily a mid- and low-latitude phenomenon. Thermocline depth and intensity vary with season, local conditions (e.g., storms), currents, and many other factors.
By contrast, the southern polar ocean is only weakly stratified. The cold temperature of southern ocean surface water closely matches that of deep water, so no thermocline divides surface water from deep water (see Figure 74-2). The absence of confining continental margins and mixing at the boundaries of the Antarctic Circumpolar Current minimize salinity differences. Turbulence and weak stratification encourage a huge volume of deep-water upwelling, which contributes to high surface nutrient levels and high biologic productivity.
Ocean Circulation
There are six great current circuits in the world ocean, two in the Northern Hemisphere and four in the Southern Hemisphere. Five are geostrophic gyres–gyres that flow around the periphery of an ocean basin: the North Atlantic gyre, the South Atlantic gyre, the North Pacific gyre, the South Pacific gyre, and the Indian Ocean gyre. Although it is a closed circuit, the sixth and largest current is technically not a gyre because it does not flow around the periphery of an ocean basin. The West Wind Drift, or Antarctic Circumpolar Current, as this exception is called, flows endlessly eastward around Antarctica, driven by powerful, nearly ceaseless westerly winds. This greatest of all the surface ocean currents is never deflected by a continent. Figure 74-3 shows the major surface currents of the world ocean.
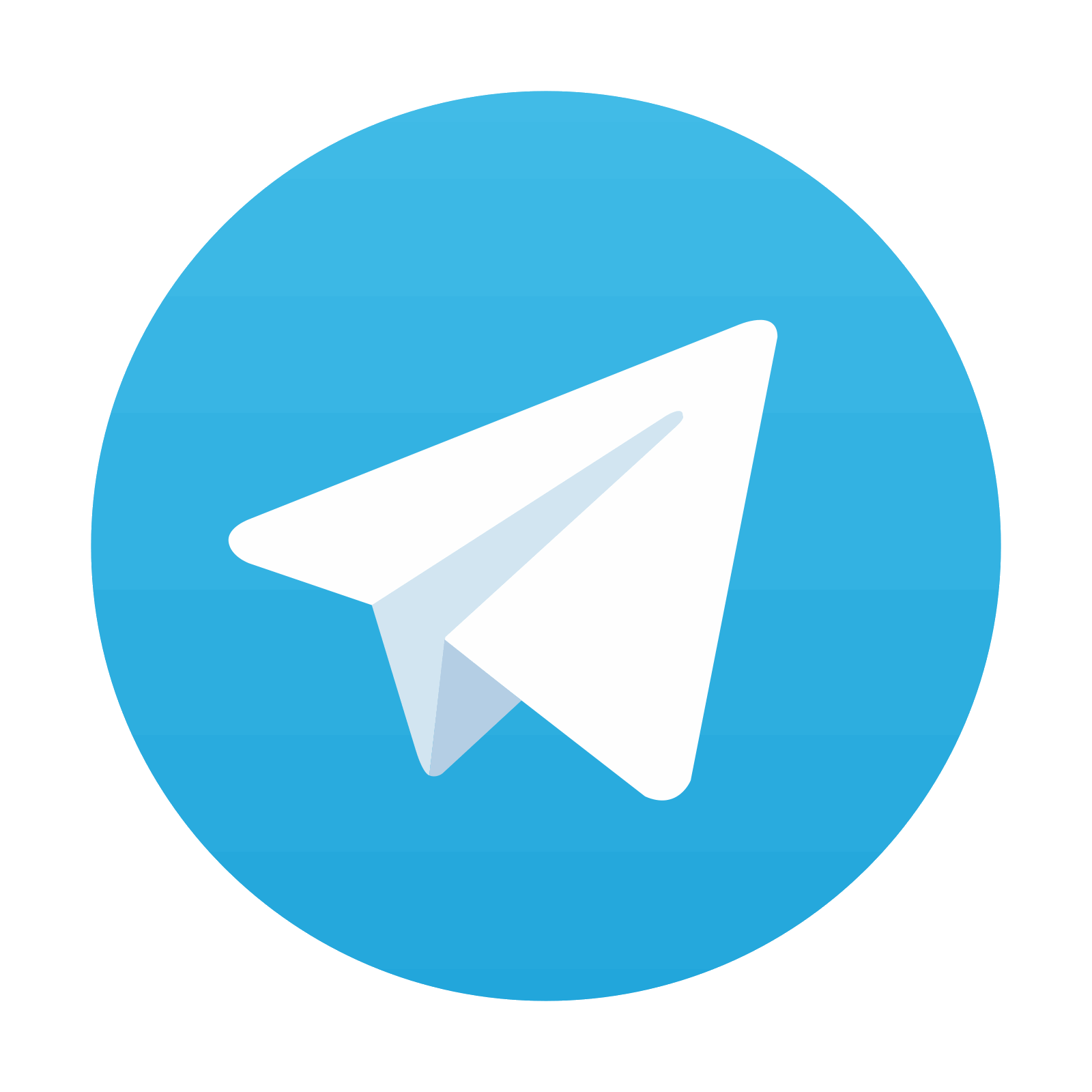
Stay updated, free articles. Join our Telegram channel
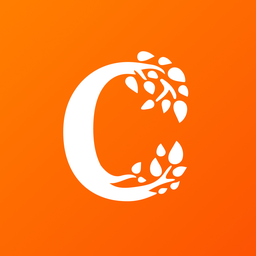
Full access? Get Clinical Tree
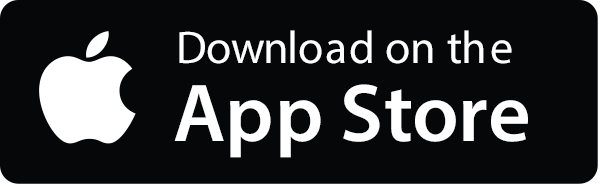
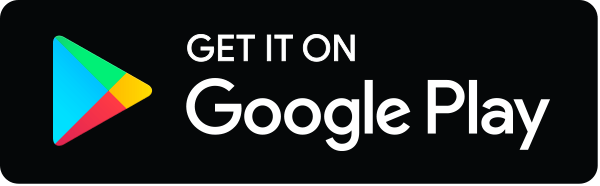