Introduction
Thermoregulation refers to the ability of an organism to maintain a set body temperature by active and passive physiologic processes. Humans are able to maintain internal equilibrium within ±0.2 °C of a set point, usually 37 °C. This ability to maintain thermal stability despite changes in ambient temperature is an important part of homeostasis.
Compared with adults, neonates and infants are more susceptible to developing hypothermia. Prior studies demonstrated a clear relationship between poor temperature regulation in the preterm newborn and increased mortality [1]. Therefore, strategies to maintain normothermia are a critical part of perioperative care.
With a focus on neonates and infants, this chapter will discuss: the physiology and pathophysiology of temperature regulation; temperature monitoring devices; and strategies to maintain thermoregulation under general anesthesia.
Normal Body Temperature
Body temperature varies with age, time of day, and level of activity, but is usually maintained within a narrow range with a mean of 37 °C (98.6 °F). When compared to older children and adults, infants and young children tend to have a higher core body temperature. In the neonatal period (0–28 days), temperatures as high as 37.5–38 °C are considered normal. These higher temperatures are attributed to the higher metabolic rates found in neonates and smaller children [2].
Hyperthermia, or fever, in neonates is defined by rectal temperature >38 °C (100.4 °F), while hypothermia is defined as core body temperature below 35 °C (95 °F). Mild hypothermia is defined as a core temperature 32–35 °C (90–95 °F); moderate hypothermia as 28–32 °C (82–90 °F); and severe hypothermia below 28 °C (82 °F) [3].
Physiology of Temperature Regulation
Thermoregulation is the body’s ability to maintain normothermia through a feedback system. The hypothalamus, which relies on afferent nerves to sense body temperature and efferent nerves to send signals to effector organs, is the central regulator that triggers actions to adjust body temperature [4].
The anterior hypothalamus receives afferent input from ascending spinal pathways via peripheral warm (via unmyelinated C-fibers) and cold (via thin myelinated Aδ fibers) receptors located in the skin, around great vessels, in the viscera, brain, and spinal cord. When the anterior hypothalamus detects deviations outside the normal temperature range, signals are sent to the posterior hypothalamus, which stimulates appropriate physiologic responses [4–6].
Input from receptors in the CNS and viscera control the majority of autonomic responses that regulate temperature. The main target organs are the blood vessels and muscles. When core temperature rises, vasodilation of blood vessels increases blood flow to the skin surface and excess heat is lost to the environment through radiation and conduction. Perspiration results in cooling through the evaporation of sweat from the skin. When core temperature falls, the body generates heat through voluntary muscle activity, called shivering. The body retains heat through vasoconstriction, which reduces blood flow to the skin and subsequently decreases heat loss to the environment [4,5].
Skin receptors only contribute about 20 percent of the input to the anterior hypothalamus. However, this input is the main driving force for behavioral responses seen in adults, who may put on/take off clothing or move to more/less warm environments. Neonates and children rely on their caregivers for behavioral modifications [6,7].
Although highly regulated mechanisms of thermoregulation are present in adults, these pathways are immature and inefficient in neonates/infants. Instead, neonates/infants have a unique method for maintaining thermoregulation – non-shivering thermogenesis.
Neonatal Temperature Regulation: Non-Shivering Thermogenesis
Despite a lack of mature mechanisms to maintain normothermia, a drop in core body temperature, or cold stress, stimulates a sympathetic surge that initiates several downstream events to help neonates/infants maintain their core body temperature – non-shivering thermogenesis, increased metabolic rate, and augmented cardiac output.
Primarily, neonates generate heat through non-shivering thermogenesis. Its main source, brown adipose tissue, is highly vascular and located in the axilla, mediastinum, between scapulae, around the internal mammary vessels, blood vessels of the neck, and the adrenal glands. The large number of mitochondria and excess triglycerides make brown adipose tissue an excellent energy source for generating heat [8].
Non-shivering thermogenesis is activated through an increase in sympathetic activity initiated by hypothermia. The sympathetic surge releases norepinephrine and thyroid stimulating hormone (TSH) from the hypothalamic ventromedial nucleus. TSH stimulates release of thyroxine (T4), which is converted to triiodothyronine (T3). T3 upregulates thermogenin, a protein that uncouples oxidative phosphorylation in brown adipose tissue. When oxidative phosphorylation is uncoupled, heat is created instead of ATP. Since up to 25 percent of cardiac output is diverted through the brown adipose tissue, the generated heat is distributed throughout the body. Infants use this method of heat generation until the second year of life.
The circulating catecholamines released during a cold stress stimulate non-shivering thermogenesis to generate heat and augment the cardiac output to dissipate heat throughout the body. These adaptive mechanisms allow the infant to maintain body temperature for a few hours, but at the expense of an increased metabolic rate and exhaustion of thermogenic reserves of glycogen and brown fat. Since most brown adipose tissue is deposited during the third trimester of pregnancy, premature and low-birth weight infants have limited thermogenic capabilities and often fare worse than full-term infants.
Mechanisms of Heat Exchange
Heat exchange with the environment occurs in humans via the skin and respiratory tract. Four different types of heat transfer are important for the anesthesiologist: radiation, evaporation, convection, and conduction. The primary source of heat loss in infants is radiation (39 percent), followed by convection (37 percent), evaporation (21 percent), and conduction (3 percent).
Radiation describes the emission of thermal energy away from the human body; it depends on the difference between skin temperature and the temperature of surrounding walls. Convection is thermal energy transfer from the body to its environment by movement of fluids. The driving force is the gradient between skin temperature and the temperature of ambient air and fluids. Evaporation is the transition of a molecule from a liquid at its surface into the gaseous phase. The energy required for this transition is drawn from thermal energy. Evaporation depends on the transepithelial/transepidermal water loss (TEWL). Conduction refers to thermal energy transfer between solid objects that are in contact. In patients, heat loss depends on the temperature difference between the patient’s skin and the surface in contact with the skin. When the temperature of the surface in contact with the patient’s skin is less than the skin temperature, heat loss occurs. Metallic surfaces have high thermal conductivity and facilitate rapid heat transfer. Table 8.1 discusses methods to avoid heat loss in neonates in the delivery room and the perioperative period [5].
Hypothermia in Neonates/Infants
Several factors make neonates and infants more susceptible to hypothermia: immature thermoregulatory mechanisms, limited glycogen and brown fat stores, and physiologic factors that accelerate heat loss. During periods of cold stress, metabolic rates may rise by two- to three-fold, leading to further heat loss and other physiologic consequences that may increase morbidity and mortality during prolonged hypothermia (Table 8.2).
Table 8.2 Effects of hypothermia on neonates/children
Cardiovascular • increased heart rate • increased cardiac output • increased vasoconstriction • increased right-to-left shunting |
Pulmonary • increased pulmonary vascular resistance • increased apnea/hypoventilation • increased hypoxia/hypoventilation/hypocapnea |
Endocrine/metabolic • 2–3-fold increase in metabolic rate • increased circulating catecholamines • metabolism of brown fat • metabolic acidosis • increased ketone production |
Renal • osmotic diuresis |
Hematologic • increased PT/PTT • inhibits normal coagulation pathways • decreased platelet function |
Immune • impaired wound healing • increased risk of infection • impaired immune function |
As discussed above, neonates/infants have immature thermoregulation mechanisms that impair their ability to maintain an appropriate core body temperature, while dramatically increasing their energy expenditure. Several anatomic and physiologic differences also contribute to their susceptibility to hypothermia. The reduced effectiveness of vasoconstriction and decreased subcutaneous fat impair their ability to retain heat, while increasing radiant and conductive heat loss to the environment. Additionally, a large skin surface area-to-body-mass ratio and a high basal metabolic rate accelerate radiant and evaporative heat loss [5,9].
When faced with cold stress, the sympathetic surge initiates non-shivering thermogenesis and augments cardiac output to maintain thermoregulation. However, lipolysis of brown fat during non-shivering thermogenesis produces ketones, which results in metabolic acidosis and osmotic diuresis. The increase in cardiac output is coupled to an increase in cardiac oxygen consumption and workload, which may exhaust the reserves of brown fat and glycogen stores during prolonged hypothermia [9].
Hypothermia is a risk factor for hypoventilation and apnea, which results in hypercapnia, hypoxia and respiratory acidosis. In conjunction with the metabolic acidosis associated with non-shivering thermogenesis, the respiratory acidosis may further increase pulmonary vascular resistance (PVR). Increases in PVR can lead to persistent pulmonary hypertension of the newborn (PPHN), when blood shunts from right to left through fetal circulatory pathways. This may lead to severe hypoxemia that does not respond to normal measures [10,11]. Additionally, increases in PVR may worsen right-to-left shunting through a patent ductus arteriosus (PDA), a ductal-dependent cardiac lesion, or nonrestrictive anatomical shunt. In the setting of the preterm neonate, an increase in right-to-left shunting through a PDA may lead to systemic hypoperfusion, tissue hypoxia, and further metabolic acidosis [9].
Although somewhat impaired in neonates, hypothermia-induced vasoconstriction may reduce tissue oxygen partial pressure and impair wound healing. Furthermore, immune functions such as chemotaxis, migration, and phagocytosis are impaired and may increase infection rates. Hypothermia may inhibit normal coagulation pathways through prolonged prothrombin/partial thromboplastin time and impaired platelet function [9].
Hypothermia in neonates has detrimental effects on almost every body system, which contributes to increased morbidity and mortality in the perioperative period: cardiac (increased heart rate, cardiac output, vasoconstriction, right-to-left shunting); pulmonary (increased PVR, apnea/hypoventilation, hypoxia); metabolic and endocrine (increased metabolic rate, circulating catecholamines, metabolism of brown fat, metabolic acidosis); hematology (increased bleeding times, prolonged PT/PTT, inhibition of platelet function); and immune (impaired wound healing and immune function, increased infection rates) [5,9]. A notable exception is medically induced hypothermia, which has been found to minimize neural damage after hypoxia and ischemia in term infants.
Thermoregulation During General Anesthesia
Operating rooms represent a specific hazard to thermoregulation in neonates/infants due to a combination of several factors: (1) anesthetic-induced inhibition of thermoregulatory mechanisms; (2) a reduction in metabolic rate under anesthesia; (3) redistribution of heat throughout the body; and (4) increased heat loss through environmental exposure and increased insensible losses [9].
Anesthetic agents significantly inhibit non-shivering thermogenesis and vasoconstriction, while decreasing metabolic rate. Opioids and propofol reduce the temperature threshold for vasoconstriction and shivering, while neuromuscular blockers completely abolish the shivering response. Although nitrous oxide has less inhibitory effect on vasoconstriction, halogenated volatile anesthetics cause vasodilation and inhibit thermoregulatory mechanisms in a dose-dependent manner. Brismar et al. demonstrated almost a 30 percent reduction in metabolic rate in patients under general anesthesia [6,12].
Patients under general anesthesia show a characteristic temporal pattern of events that influences body temperature. Induction of general anesthesia leads to vasodilation and triggers phase 1, called internal redistribution. Within the first 30–45 minutes, the central core temperature drops by 0.5–1.5 °C. Importantly, the overall heat balance of the body only slightly decreases as the majority of energy is not lost to the environment but rather redistributed to the periphery and skin. In the first hour after induction, redistribution accounts for 81 percent of the temperature decrease. The second phase, termed thermal imbalance, lasts 2–3 hours and is characterized by a decrease in core body temperature of about 0.5–1.0 °C per hour. Thermal imbalance reflects a combination of reduced heat production (decreased metabolic rate, decreased muscle activity) and increased heat loss to the environment (radiation, evaporation, conduction, convection). During the third phase, thermal steady state, the decreased core body temperature causes a drop in the heat gradient between body and environment. At this point, the dissipation of heat equals heat production and the core temperature remains constant (usually between 34.5 and 35.5 °C) [9].
As a result of anesthetic-induced vasodilation and inhibited thermoregulatory mechanisms, neonates/infants are even more vulnerable to environmental heat loss in the operating room. Due to their high surface area-to-body-mass ratio, decreased subcutaneous fat, and high metabolic rate, rapid heat loss occurs through (1) radiant heat loss to the cold operating room; (2) conductive heat loss from cold intravenous fluids or blood products; and (3) evaporative heat loss from exposed viscera and mechanical ventilation. In an awake infant, heat loss to the environment is offset by a high metabolic rate. Since vasoconstriction, increases in metabolic rates, and non-shivering thermogenesis are the primary responses available to anesthetized infants, blunting of these responses can lead to profound hypothermia under general anesthesia [9].
Short procedures can lead to significant heat loss when the proper preventive steps are omitted, while lengthy surgeries can easily overwhelm the limited capabilities of newborns/infants to maintain adequate body temperature. Therefore, it is important for the anesthesiologist to take appropriate measures to reduce perioperative heat loss and maintain normothermia. Table 8.1 provides a summary of interventions to address perioperative heat loss. In brief, the following steps should be considered for every surgery: (1) warming of the operating room prior to patient arrival; (2) use of radiant warmers and heating pads/mattresses; (3) institution of closed-circuit anesthesia, with use of humidified/warmed gases; (4) warming of intravenous infusions/irrigation fluids; (5) use of forced-air warming blankets to cover body areas not involved in the surgical procedure; and (6) keeping the patient covered by blankets/clothes [5,13].
Anesthesiologists most often employ passive rewarming methods, which include forced-air warming devices and warm blankets. However, it is important to note that these techniques deactivate skin cold receptors and abolish the body’s own thermoregulatory functions. This contributes to worsening hypothermia if passive surface rewarming is unable to maintain normothermia [9]. Forced-air warming devices have been associated with third degree thermal burns in infants. It is crucial that they be used according to manufacturers’ recommendations, and caution should be used when using the highest temperature setting [14].
In summary, general anesthesia increases the risk of perioperative hypothermia in neonates and infants. Intraoperative hypothermia leads to several adverse effects in the perioperative period: (1) increased metabolic rate and oxygen consumption; (2) exhaustion of thermogenic reserves; (3) diminished metabolism of anesthetic agents resulting in prolonged opioid effects and neuromuscular blockade; (4) increased bleeding risk due to inhibition of normal coagulation pathways; (5) increased risk of wound infections. This underlines the importance of meticulous intraoperative temperature maintenance by addressing the various heat loss mechanisms [4].
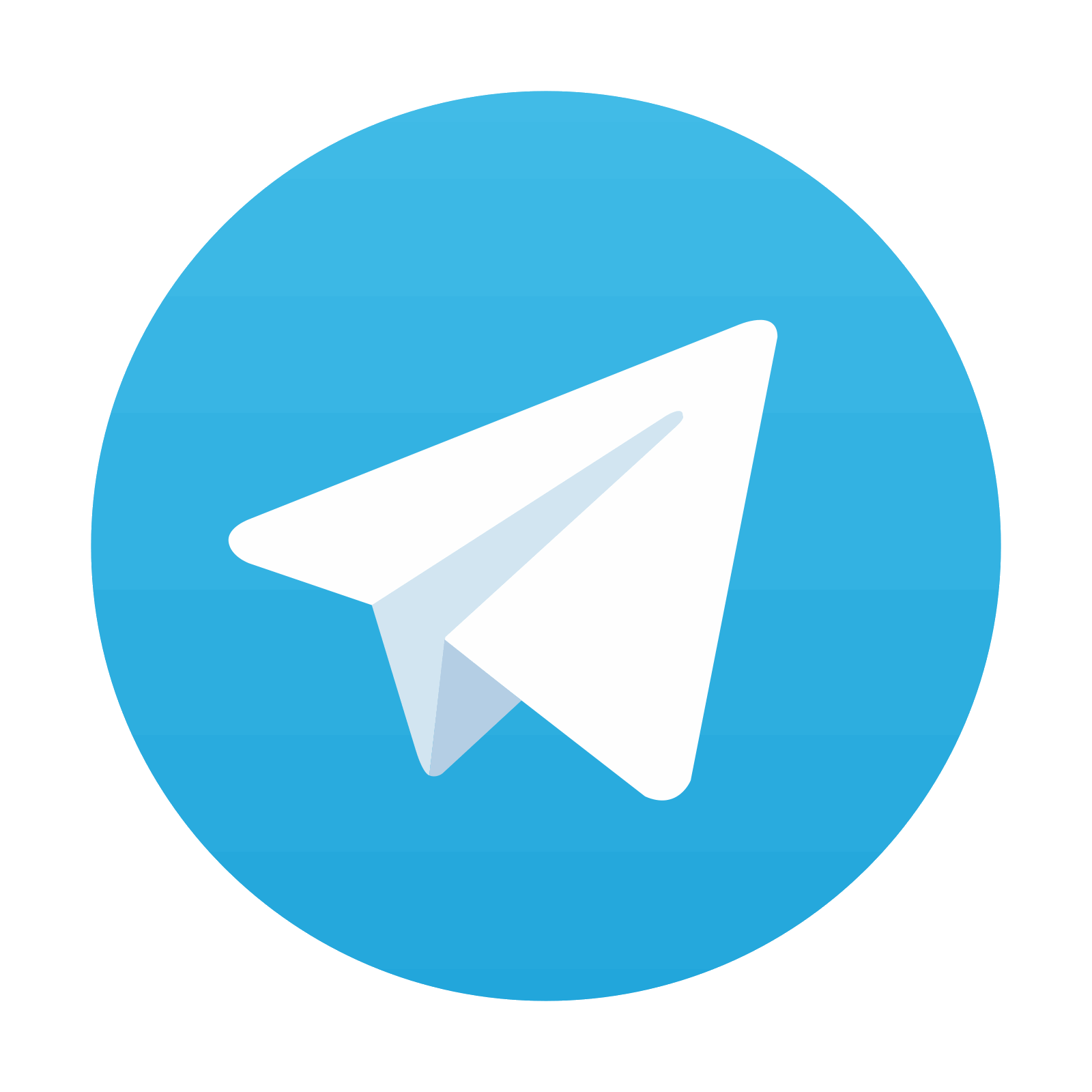
Stay updated, free articles. Join our Telegram channel
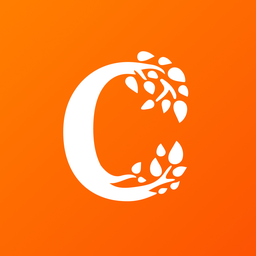
Full access? Get Clinical Tree
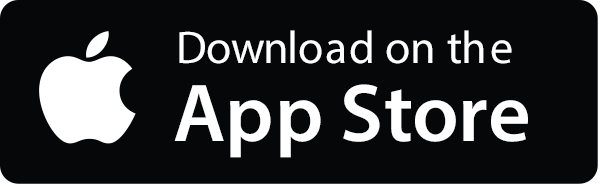
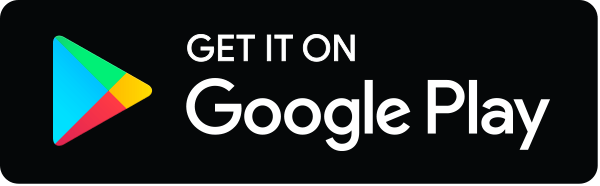