Glucose Homeostasis in Neonates
Under physiologic conditions, glucose levels are tightly controlled. Glucose loading stimulates insulin, which in turn stimulates peripheral glucose uptake, glycogen synthesis, and lipogenesis while also inhibiting processes that produce glucose. Fasting causes an increase in counter-regulatory hormones, including glucagon, catecholamines, cortisol, and growth hormone, which act to stimulate glucose production and decrease synthesis of glucose storage molecules. Early in a fast, euglycemia is maintained primarily by glycogenolysis. During a prolonged fast or in situations in which glycogen stores are limited, gluconeogenesis, lipolysis, and ketogenesis are more important. Amino acids from muscle catabolism and glycerol generated by lipolysis are substrates for gluconeogenesis. Lipolysis also produces free fatty acids, which are substrates for ketogenesis. Ketones can be used as an alternative fuel source by muscle to preserve adequate glucose for the brain and erythrocytes. Therefore, normal glucose homeostasis requires intact metabolic and endocrine pathways as well as adequate hepatic glycogen, muscle, and fat stores to drive endogenous energy production during times of fasting [1].
Neonates and small infants have a number of metabolic differences from older children and adults that affect glucose homeostasis. The fetus receives a continuous supply of glucose through placental blood flow. At birth, glucose levels abruptly decrease and subsequently increase over 1–2 hours due to increasing counter-regulatory hormones. Glucose levels stabilize as gluconeogenic and ketogenic pathways mature and oral glucose intake improves over the next 24 hours [2,3]. The endogenous glucose production rate in neonates is 4–6 mg kg–1 min–1, but is dependent on exhaustible glycogen, muscle, and fat stores [3]. Up to 80 percent of energy requirements are supplied by fat metabolism as glycogen stores fall [4]. Fetal weight doubles between 32 weeks gestational age and term, mostly due to hepatic glycogen and adipose deposition [2]. Therefore, neonates that are premature or small for gestational age have significantly decreased metabolic reserve. Neonates also have higher energy requirements compared to adults. Glucose consumption in neonates is 6 mg kg–1 min–1 compared with 1–2 mg kg–1 min–1 in adults. This is primarily due to the increased brain-to-body size ratio and resulting increased cerebral glucose utilization in children [1,2].
The combination of increased metabolic rate and decreased energy reserves increases the risk of hypoglycemia in neonates. The presentation of hypoglycemia is nonspecific and includes autonomic symptoms (diaphoresis, tremor, and tachycardia) from increased catecholamine secretion and neuroglycopenic symptoms (altered mentation, lethargy, hypotension, and seizures) due to decreased cerebral glucose supply [1]. Multiple studies have shown an association between neonatal hypoglycemia and brain injury, as measured by structural abnormalities on radiologic studies and long-term neurodevelopmental abnormalities [1,5–7]. However, some studies have found no such correlation [8]. No study has determined a duration, severity or number of hypoglycemic episodes that is harmful to neonates and the contribution of hypoglycemia independent of other factors, such as hypoxia-ischemia, has not been proven [3]. Possible mechanisms for hypoglycemic cerebral injury include increased cerebral blood flow with decreased autoregulation due to the hormonal stress response and alterations in brain metabolism, neurotransmitters, and acid–base balance [9]. Glucose is the brain’s primary fuel source. However, in contrast to adults, the neonatal brain also uses alternative energy sources, such as ketone bodies and lactate, under physiologic conditions. The presence of alternative fuels is therefore protective against hypoglycemic brain injury and their availability must be taken into account when assessing the severity of neonatal hypoglycemia. In patients with impaired metabolic responses, such as those with abnormal metabolic pathways, decreased substrate availability or insulin-driven hypoglycemia, mild hypoglycemia is more dangerous than in otherwise healthy patients [2,3,5,10]. This ability to use alternative fuels is a confounding factor in that it makes it difficult to define hypoglycemia in neonates.
Most studies use a threshold of 40–45 mg dl–1 (2.2–2.6 mmol L–1) to define neonatal hypoglycemia, though the threshold for intervention may be higher, especially in neonates with impaired metabolic responses [1–3]. However, glucose values in this range may occur normally in healthy, full-term neonates in the first 48–72 hours of life. Patients with increased risk for developing hypoglycemia include premature and small neonates, those with increased metabolic demands due to physiologic stressors, and patients with inappropriately elevated insulin levels or counter-regulatory hormone deficiency. Common physiologic stressors include sepsis, hypothermia, and hypoxia. Inappropriate insulin elevation can be iatrogenic, due to insulin administration or an abrupt discontinuation of glucose-containing fluids (including parenteral nutrition). Transient insulin elevation is also seen in neonates born to mothers with diabetes or those who received dextrose-containing fluids immediately prior to or during delivery. Sustained insulin elevations are seen in some endocrinopathies. Counter-regulatory hormone deficiency is seen in hypopituitarism, adrenal insufficiency, and patients receiving beta blockers [2,3,11].
Maintenance glucose administration in neonates is typically started at 4–7 mg kg–1 min–1 to match endogenous production. This is commonly supplied as 10 percent dextrose, requiring infusion rates of 60–100 ml kg–1 day–1 (2.5–4 ml kg–1 h–1). Patients with high volume requirements, such as extremely low birth weight infants, may require more dilute solutions to avoid hyperglycemia, while those who are fluid restricted may require more concentrated solutions to avoid hypoglycemia. Concentrations greater than 12.5 percent are rarely required, but should be given centrally to avoid sclerosis of peripheral veins or tissue damage from infiltration [3,12]. Neonates undergo a postnatal diuresis in the first few days of life that results in loss of 10–15 percent of body weight as water. This is a normal physiologic process and patients in whom this process is prevented by volume expansion have poor outcomes. Therefore, patients may require concentrated dextrose infusions to meet nutritional requirements for the first few days of life [13,14]. Severe or symptomatic hypoglycemia requiring immediate intervention may be treated with a bolus of 3–5 ml kg–1 of 10 percent dextrose (300–500 mg kg–1) followed by an infusion [3].
Hyperglycemia is a common finding in critically ill patients that can also complicate the management of neonates. Physiologic stressors, such as acute illness, pain, or surgery disrupt normal glucose homeostasis by increasing counter-regulatory hormones and inducing insulin resistance. This results in inappropriate endogenous glucose production as well as decreased peripheral glucose uptake. Neonates that are premature or small for gestational age also have impaired glucose homeostasis at baseline. Iatrogenic factors, such as administration of dextrose-containing fluids (including parenteral nutrition and blood products), catecholamines, and corticosteroids, also contribute to hyperglycemia [1–3,15]. The detrimental effects of hyperglycemia and benefits of glucose control are established in some adult populations. Blood glucose abnormalities, including hypo- and hyperglycemia as well as glucose variability, have been associated with increased morbidity and mortality in a number of pediatric studies [16–18]. However, hyperglycemia is only a prognostic indicator in these studies. A directly causative effect of hyperglycemia on patient outcomes has not been proven [18] and the benefits of glucose control with insulin have not been established in pediatric patients due to increased risk of hypoglycemia [1,18].
Hyperglycemia is commonly defined as glucose greater than 126 mg dl–1 (7 mmol L–1), with treatment generally indicated above 180 mg dl–1 (10 mmol L–1) [1–3]. Hyperglycemia causes glycosuria and osmotic diuresis, which can result in significant volume depletion and electrolyte imbalances, especially in small infants [2,19,20]. Glycosuria may be present even with minimal hyperglycemia in preterm neonates who have a low renal threshold for glucose reabsorption [3,11,21]. There have been concerns that hyperglycemia can lead to significant fluid shifts with resultant cerebral pathology and neurodevelopmental effects, though there is no evidence that self-limited hyperglycemia below 360 mg dl–1 (20 mmol L–1) has significant effects in the absence of osmotic diuresis [3]. Hyperglycemia in the presence of hypoxia is associated with worsening of neurologic outcomes in adult and animal studies. This is due to anaerobic glycolysis of excess glucose with resultant intracerebral lactic acidosis and compromised cellular function [9,10]. In contrast, moderate hyperglycemia may not worsen, and actually may be protective from, ischemic damage in neonates due to differences in glucose and lactate metabolism in the neonatal brain [9–11,19,22]. Hyperglycemia in neonates is generally self-limited and related to iatrogenic factors or underlying medical comorbidities. Treatment involves limiting glucose administration and treating the underlying illness. Rarely, insulin is required and may be started at 0.03–0.05 units kg–1 h–1 [3,12].
Perioperative Glucose Management
Fasting, surgical stress, and anesthesia have significant effects on glucose homeostasis in the perioperative period. These effects are well-studied in pediatric patients, but studies including or focusing on neonates are limited. As noted in the previous section, neonates have significant metabolic differences from older children that may limit the applicability of pediatric studies to the neonatal population.
Hypoglycemia in healthy, fasting children presenting for surgery is rare (less than 2.5 percent) and the incidence has decreased as more liberal fasting guidelines, including intake of clear fluids until two hours preoperatively, have been implemented [18–21]. Most children who were hypoglycemic had very prolonged fasting times [19]. Children who underwent daytime fasting for afternoon procedures had lower glucose levels than children who fasted overnight for morning surgery, independent of the fast duration, due to diurnal variations in cortisol secretion [23]. In infants and young children, preoperative glucose levels did not correlate with age, weight, or duration of the fast [24]. Studies of fasting neonates have demonstrated that neonates under one week of age can maintain euglycemia without intraoperative glucose administration [4,25,26]. In one study, neonates of normal birth weight were able to maintain euglycemia for up to seven days without glucose administration due to increased fat mobilization [4]. However, hypoglycemia did occur in patients under 48 hours old or who were receiving preoperative glucose infusions that were stopped intraoperatively [25,26]. Preoperative oral and intravenous glucose loading raises insulin levels and can cause rebound hypoglycemia if glucose infusions are stopped intraoperatively [25].
Intraoperative increases in glucose due to surgical stress have been demonstrated in multiple pediatric studies [21]. Neonatal patients also mount an endocrine and metabolic stress response to surgical stimulation. Operative trauma in neonates results in an increase in oxygen consumption, energy expenditure, and blood glucose levels that persist until 12–24 hours postoperatively [4,27,28]. Glucose elevations are mediated by an early rise in catecholamines, followed by sustained cortisol and glucagon elevations. Insulin secretion remains low until 12–24 hours after surgery, with longer suppression in premature infants, who remain hyperglycemic longer in the postoperative period [27–29]. Increasing levels of surgical stress are associated with greater and more prolonged metabolic changes and worse outcomes [30]. In neonates, energy required for surgical trauma is diverted from energy used for growth. This avoids the overall increase in energy expenditure seen in adults, but does result in retarded neonatal growth during times of metabolic stress [27].
Anesthesia reduces the energy requirements of children up to 50 percent from their awake values [31,32]. The combination of reduced energy requirements and increased serum glucose due to impaired metabolism decreases the amount of glucose administration required intraoperatively [13]. However, the type of anesthetic also plays a role in determining intraoperative glucose requirements. In neonates and children, neuraxial anesthetics significantly decrease the hormone stress response compared to opioids [33,34] and volatile anesthetics [35,36]. Opioids, in turn, provide better suppression than volatile anesthetics [37]. Suppression of the surgical stress response blunts the normal intraoperative rise of glucose and places patients at risk for hypoglycemia. Consideration should be given to monitoring glucose levels in patients receiving neuraxial anesthetics [35].
The goals for intraoperative fluid management are to maintain adequate circulating volume to provide organ perfusion and oxygen delivery and to maintain electrolyte and glucose homeostasis [13]. Neonates are a particularly challenging group of patients in which to maintain these goals, as their volume, electrolyte, and glucose requirements are markedly different from older patients and change throughout the neonatal period. Maintenance volume requirements as determined by Holliday and Segar are approximately 4 ml kg–1 h–1 in neonates [32]. Most neonates presenting for surgery from the NICU are well-resuscitated and receiving glucose-containing fluids. However, fasting neonates not receiving infusions will have a fluid deficit. In addition, surgical trauma requires additional fluid administration. Berry proposed administration of 15–20 ml kg–1 of fluid over the first hour to correct volume deficits, followed by 6 ml kg–1 h–1 for maintenance fluids and an additional 2–6 ml kg–1 h–1 to cover surgical trauma [21]. Many studies employ fluid algorithms similar to that proposed by Berry. In some studies, glucose-containing solutions were administered at these high rates to cover maintenance requirements and replace fasting deficit and insensible loss without other crystalloid administration. It is therefore important to consider both the concentration of fluid administered and the rate at which it is given to determine the total glucose load in these studies.
Administration of 5 percent dextrose infusions to infants and young children causes hyperglycemia intra- and postoperatively. In most studies, the glucose rose intraoperatively whether or not dextrose was administered, and higher concentrations of dextrose were associated with increasing frequency of hyperglycemia. This has resulted in a trend toward using low glucose concentrations (1–2.5 percent dextrose) or avoiding glucose administration in these patients [15,19–21,38]. However, it should be noted that maintenance of euglycemia does not imply that patients have adequate glycogen stores for a prolonged intraoperative fast. In one study, infants under one year old not receiving glucose had an average increase in their glucose levels, but 40 percent of patients failed to increase or had a decrease in the glucose levels within normal limits. These patients required lipolysis to maintain euglycemia due to inadequate glycogen stores [39].
Studies assessing the need for intraoperative glucose administration in neonates are limited. Most neonates who did not receive intraoperative glucose were able to maintain euglycemia [25,26]. However, hypoglycemia was found in neonates less than 48 hours old or in whom preoperative glucose infusions were discontinued intraoperatively [25]. In addition, fat mobilization was required to maintain euglycemia in the fasting neonates due to limited glycogen stores [26]. Glucose administration at 100 mg kg–1 h–1 maintains euglycemia in neonates, though some patients did have declining glucose levels within the reference range [40]. Administration of 120–300 mg kg–1 h–1 of glucose is sufficient to prevent lipid mobilization and to allow glucose levels to rise without causing hyperglycemia in young infants [39] and neonates [26]. These rates are similar to the rates of endogenous glucose production in neonates (240–360 mg kg–1 h–1). However, hyperglycemia may still occur with these regimens during longer procedures. Higher rates of glucose administration resulted in hyperglycemia [25,41].
Given the risks of undetected hypoglycemia, intraoperative glucose administration is warranted in neonates. Neonates at particular risk include patients who are premature or small, less than 48 hours old, receiving glucose-containing solutions preoperatively, undergoing long procedures (2–3 hours), or who have a neuraxial block. Glucose can be supplied intraoperatively with D10% at a low infusion rate (1–3 ml kg–1 h–1) or with more dilute solutions close to normal maintenance rates. Separate, non-glucose-containing fluids should be used for boluses to replace the fasting deficit and intraoperative losses. Patients receiving glucose-containing fluids preoperatively require intraoperative glucose administration to prevent rebound hypoglycemia, though the rate can be decreased in anticipation of the surgical stress response. Glucose should be periodically monitored in neonates, especially for long procedures or if glucose-containing fluids are being withheld.
References





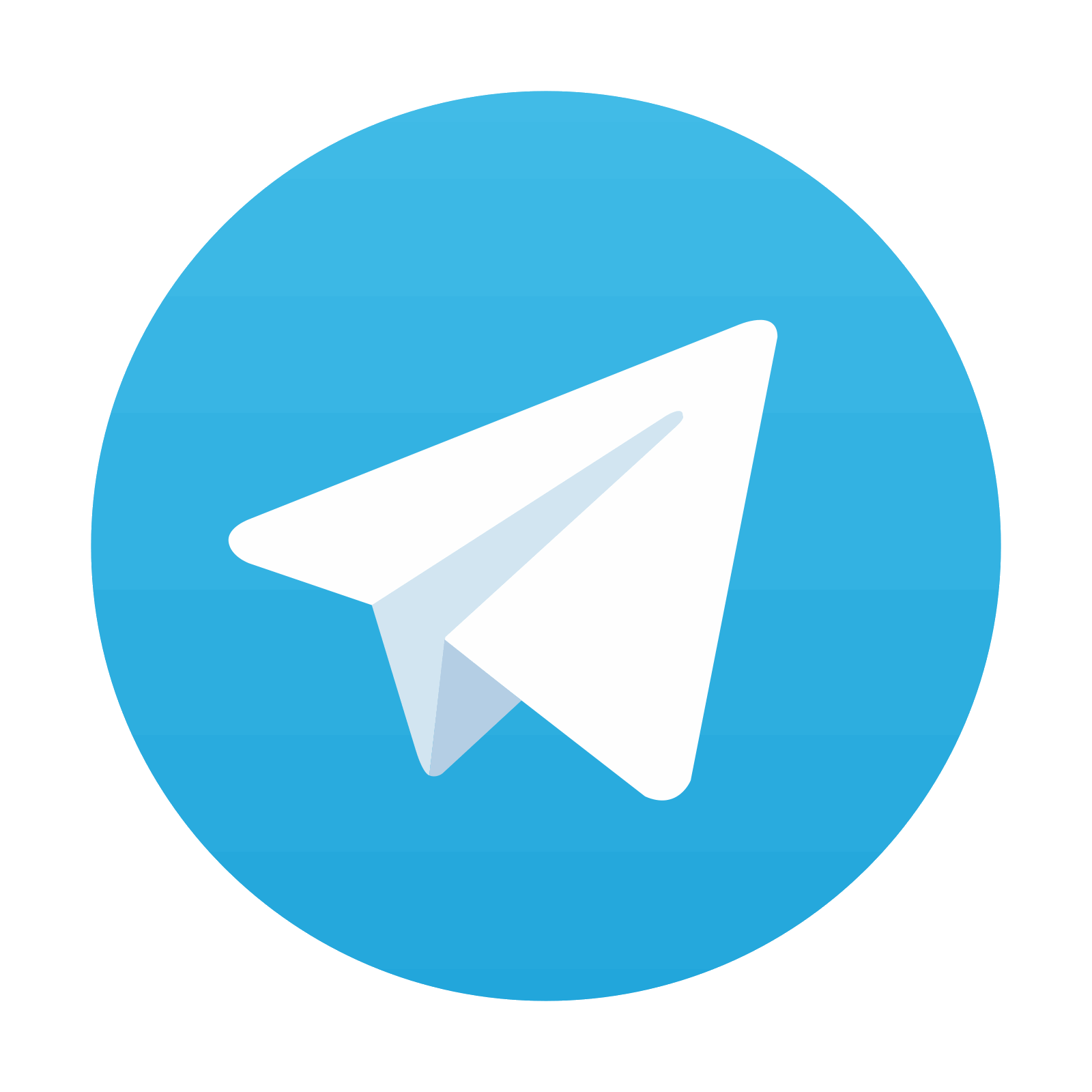
Stay updated, free articles. Join our Telegram channel
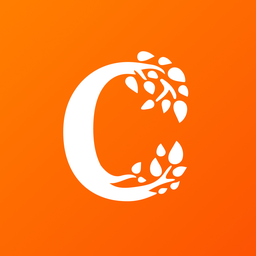
Full access? Get Clinical Tree
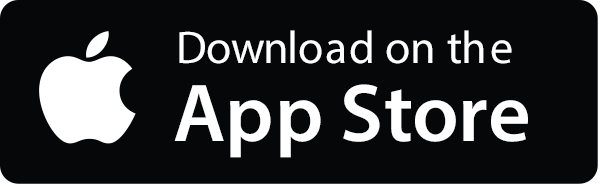
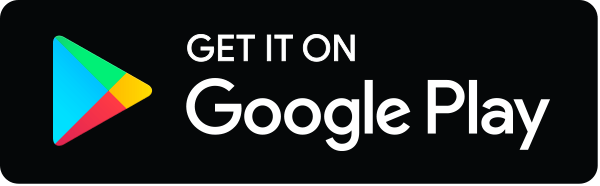
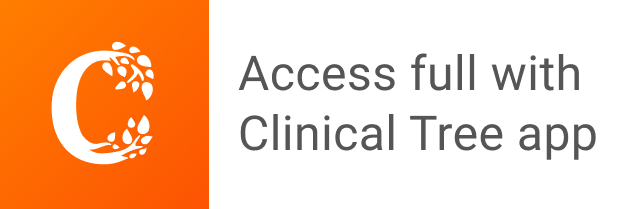