Arterial carbon dioxide partial pressure (PaCO2) is an essential indicator of ventilation and respiratory function. PaCO2 represents the balance between production and elimination of carbon dioxide, and must be maintained within acceptable limits to ensure optimal acid–base homeostasis and health. This chapter reviews the basic physiology, interpretation, indications, and limitations of the two most common forms of noninvasive CO2 monitoring – capnography and transcutaneous CO2 monitoring – and will also review optimal PaCO2 targets and the effect of hypocapnia on cerebral perfusion.
Noninvasive Carbon Dioxide Monitoring
Arterial carbon dioxide partial pressure is an essential indicator of ventilation and respiratory function. Measurement of PaCO2 through arterial blood gas (ABG) analysis is the gold standard for assessing ventilation. Unfortunately, measurement of PaCO2 is invasive. Perhaps more importantly, PaCO2 can be a dynamic and rapidly changing value. To overcome such problems, noninvasive monitors are used to provide a continuous estimate of PaCO2. The two most primary noninvasive monitoring methods include capnography and transcutaneous monitors.
Capnography
Capnography refers to the noninvasive measurement of the partial pressure of carbon dioxide in exhaled breath, expressed as the CO2 concentration over time [1,2]. The relationship of CO2 concentration to time can be represented graphically as a waveform or capnogram, or can be used to determine the maximum CO2 concentration at the end of each tidal breath, or end-tidal CO2 (EtCO2). Capnography has been widely utilized in the United States since the 1980s, and has many clinical uses, including the assessment of disease severity or response to therapy, or the confirmation of proper endotracheal tube (ETT) placement.
Capnography devices can be categorized based on their location for sampling, and therefore also on the types of patients for whom they are effective. Diverting capnometers are known as sidestream systems, and nondiverting capnometers are known as mainstream systems. Sidestream devices transport a sample of a patient’s respired gases from the sampling site, through a sampling tube, to the sensor at a site distant from the sample site. Mainstream devices do not transport gas away from the sampling site, and therefore measure CO2 at the actual sample site [3,4].
Mechanism of CO2 Detection: Capnography
Capnography uses infrared (IR) radiation and absorption to detect CO2. Molecules of CO2 absorb IR radiation at a very specific wavelength (4.26 µm). CO2 monitors use lightweight IR sensors to emit IR light through adapter windows to a photodetector, which is sensitive to the IR band of CO2. The detector is located on the other side of the airway adapter. The light that reaches the photodetector is used to measure EtCO2. The IR radiation absorption is highly correlated with the CO2 concentration present. Therefore, measurement of the IR radiation at the specific wavelength in a breath sample allows determination of CO2 levels in that sample.
Mainstream Versus Sidestream Capnographs
Mainstream devices measure CO2 directly from the airway, with the sensor located on the airway adapter at the hub of the ETT, between the breathing circuit and the ETT. Mainstream capnography does not require gas sampling, as the measurement is made directly in the airway. Mainstream sensors are heated to slightly above body temperature to prevent condensation of water vapor, since this can cause falsely high CO2 readings.
In mainstream capnography, a source emits IR that includes the absorption band for CO2. The CO2 within the exhaled gas in the circuit preferentially absorbs the radiation at its specific wavelength and passes other wavelengths. Photodetectors on the other side of the airway adapter measure the transmitted radiation. The detected signals are then amplified and transmitted via cable to a monitor where the partial pressure of the CO2 is calculated and displayed.
In contrast, sidestream devices measure CO2 via nasal or nasal–oral cannula by aspirating a small sample from the exhaled breath through cannula tubing to a sensor located inside the CO2 monitor. This provides a unique advantage, allowing monitoring of non-intubated subjects, since sampling of the expiratory gases can be obtained from the nasal cavity. Gases can even be sampled from the nasal cavity during the administration of oxygen in patients receiving simultaneous oxygen administration using nasal cannula. Sidestream devices may require additional safeguards, including a gas scavenging system to collect anesthetics gases in the sample, and a water trap to collect condensation from humidified sample gas or patient secretions. Differences between mainstream and sidestream capnograms are summarized in Table 5.1.
Table 5.1 Comparison of mainstream and sidestream carbon dioxide analyzers
Mainstream | Sidestream | |
---|---|---|
Location of infrared analysis unit | At airway connector | In the monitor |
Use on extubated patients | No | Yes |
Durability | Durable | Variable |
Sample volume drawn | None | Low volume sample |
Delay between sampling time and waveform display | None | Less than three seconds |
Sensor 10–90 percent rise time | <70 milliseconds | >200 milliseconds |
Waveform display | Crisp | Smooth appearance |
Accuracy of waveform shape | Excellent | Variable |
Changes in water vapor pressure | Not affected | Affected due to condensation and drying of sample |
Potential disadvantages of mainstream capnography include relative fragility of adapters, increased mechanical dead space, additional weight on the airway, and use limited to intubated patients. Sidestream capnography has different downsides. Measurement of CO2 distant from the sampling site can potentially be affected by variation in humidity and temperature between the sampling and measurement sites, and pressure drops through the tubing [5,6]. In addition, using a remote location for measurement can result in a delay time of up to several seconds.
Sidestream gas analyzers continuously aspirate sample gas from the breathing circuit at variable sample flow rates. Low-flow sidestream systems usually utilize flow rates of approximately 50 ml min–1 and are used for patients with low tidal volumes (i.e., neonates, infants, or patients of any age with low tidal-volume breathing). One advantage of such systems is that they have less dilution from supplemental oxygen compared to high-flow systems. High-flow systems utilize flow rates closer to 150 ml min–1, but are less accurate in infants or patients with low tidal-volume breathing [7–9].
Accuracy of CO2 Detection by Capnography
Accurate assessment of CO2 levels by capnography requires sampling of an accurate breath sample, reflective of a breath sample from the lower airways. In healthy lungs, there is limited anatomical or physiologic dead space, so the differences between EtCO2 and PaCO2 are relatively small. Patients with significant lung disease may have increased physiologic dead space and issues such as ventilation–perfusion mismatch, which may influence the correlation of the EtCO2 with PaCO2 [10,11]. For such patients, the EtCO2 may only be useful for assessing trends in ventilatory status over time; isolated EtCO2 values may or may not correlate with the PaCO2.
Indications for Capnography
Capnography has many potential clinical applications. The most common include:
(1) confirmation of ETT placement;
(2) assessment of trend of respiratory status (improvement versus maintenance versus deterioration) due to progression of clinical course or response to therapy;
(3) assessment of effectiveness of resuscitation in circulatory arrest.
Endotracheal Tube Placement
Capnography has become a gold standard in the accurate determination of proper placement of an ETT (or any other advanced airway devices, like laryngeal mask airways) into the trachea [12–15]. Capnographic assessments may be quantitative, providing a numerical assessment of EtCO2, or qualitative, which uses a colorimetric display to show a range of EtCO2 values. Colorimetric capnography utilizes specially treated litmus paper that changes color when exposed to CO2 [16,17]. Purple indicates EtCO2 <3 mmHg, tan indicates EtCO2 = 3 –15 mmHg, and yellow indicates EtCO2>15 mmHg. Proper placement of an ETT in the trachea will therefore change the color of the litmus paper from purple to yellow. A monitor whose litmus paper remains purple has several possible explanations: (a) improper placement of the ETT into the esophagus; (b) proper tracheal placement with inadequate pulmonary blood flow; (c) obstruction of the ETT or airway just distal to the ETT; or (d) technical malfunction of the monitor or tubing
Capnography has proven to be superior in sensitivity and specificity to other methods of ETT placement confirmation [12], including condensation in the tube [18], rise of the chest wall with breaths [19], and assessment of breath sounds [20]. Capnography may be slightly less sensitive in patients with circulatory failure, but remains highly accurate in determining ETT location. EtCO2 should be the primary means to confirm tracheal placement of an ETT; the limitations of clinical methods indicates that these may be used adjunctively, but not primarily.
Effectiveness of CPR
EtCO2 reflects pulmonary blood flow during cardiac arrest, since alveolar ventilation and metabolism are otherwise constant. EtCO2 can therefore be used as a noninvasive measure of cardiac output during cardiopulmonary resuscitation (CPR) [21]. Since effective CPR leads to improved cardiac output, the increase in perfusion will be reflected in a rise in the measured EtCO2 [22].
Transcutaneous Carbon Dioxide Monitoring
Transcutaneous CO2 (PtcCO2) devices provide another option for the continuous noninvasive estimation of PaCO2. Rather than sample exhaled gas, PtcCO2 monitoring uses skin sensors to calculate CO2 through biochemical assessment of pH. This therefore provides an additional option for patients who are not intubated.
Mechanism of CO2 Detection: Transcutaneous Carbon Dioxide Monitoring
PtcCO2 estimates the PaCO2 through electrochemical measurements of CO2 gas diffusing through body tissue and skin by a sensor at the skin surface by measuring the pH of an electrolyte solution that is separated from the skin by a permeable membrane [23,24]. The sensor is warmed to induce a local hyperemia, approximately 42–43 °C, which induces vasodilation of the dermal capillary bed below the sensor, increasing arterial blood flow. This vasodilation also facilitates diffusion of CO2. PtcCO2 is often slightly higher than the corresponding measured PaCO2 value. This is likely due to two main factors. First, the elevated temperature alters the solubility of CO2. Second, the hyperemia increases the metabolism of the skin cells, which contributes to CO2 levels. The PtcCO2 may therefore require a corrective algorithm to more closely align monitor values with the PaCO2 [25,26].
Transcutaneous monitoring is considered a safe procedure; however, tissue injury may occur at the measuring site, including blisters, burns, and skin tears. Since the technology involves hyperemia where the probe is applied, continuous monitoring is generally avoided, since skin-related complications primarily occur when the PtcCO2 is left in place for long periods of time. Some patients are not good candidates for PtcCO2, including patients with poor skin integrity or adhesive allergy [27,28].
Besides the safety considerations, there are some clinical situations that may lead to an increased discrepancy between the PtcCO2 and PaCO2. These may include improper probe placement or application, factors associated with variable distance from probe to capillaries (such as body wall edema or thickness of the patient’s skin or subcutaneous tissue), poor perfusion of the site of probe placement, or hyperoxia (PaO2 > 100 torr) [24,27,28].
Indications for Transcutaneous CO2 Monitoring
Like capnography, PtcCO2 assessment has many potential clinical applications. The most common include:
(1) assessment of trend of respiratory status (improvement versus maintenance versus deterioration) due to progression of clinical course or response to therapy;
(2) assessment of tissue perfusion;
(3) monitoring of acid–base status.
Evaluation of Efficacy of Ventilation During Respiratory Failure
In some cohorts of patients, PtcCO2 has been shown to be superior to capnography [27,28]. In particular, infants with congenital heart disease may have shunt or ventilation–perfusion mismatch that make EtCO2 less accurate, but can benefit from PtCO2 [29,30].
Assessment of Tissue Perfusion
Several studies have looked at the use of PtcCO2 to assess tissue perfusion in partial-thickness skin grafts for burn injury [31], and to evaluate changes in tissue perfusion during aortic cross-clamping for repair of coarctation of the aorta [32]. These studies suggest that PtcCO2 may be useful in other clinical scenarios to noninvasively assess tissue perfusion.
Monitoring of Acid–Base Status
Given the relationship of PaCO2 to pH and serum bicarbonate, it is possible that changes in PaCO2 can be used to reflect changes in pH. For example, in patients with diabetic ketoacidosis, PtcCO2 may be utilized to follow the changes that occur during treatment and resolution of metabolic acidosis [33].
Benefits and Limitations of PtCO2 Compared to EtCO2
Compared with EtCO2 monitoring, PtcCO2 monitoring requires a longer preparation time. Prior to placement of the sensor, a calibration period of approximately 2–5 minutes is required. This is followed by another equilibration period of comparable duration after placement of the probe on the patient to allow for an equilibration between the PtcCO2 and PaCO2 values.
PtcCO2 monitoring has been shown to be comparably accurate to capnography in patients with normal respiratory function and potentially more accurate in patients with congenital heart disease or ventilation–perfusion inequalities. PtcCO2 monitoring also allows monitoring of PaCO2 for patients on high-frequency oscillatory ventilation or non-intubated patients, for whom EtCO2 is more challenging, and also allows monitoring metabolic status during treatment of acidosis.
In summary, both PtcCO2 and capnography monitoring provide easily obtained, interpretable data that are within agreement of each other. Each method has unique advantages and limitations, but both provide important data that can assist in the optimal care of patients with a variety of pulmonary and metabolic diseases.
Optimal Target PaCO2
The optimal levels of PaCO2 during anesthesia and controlled ventilation depends significantly on the underlying disease process and the potential risk of mechanical ventilation required to achieve optimal gas exchange.
The PaCO2 is an indicator of CO2 production and elimination: for a constant metabolic rate, the PaCO2 is determined entirely by its elimination through ventilation. A normal value of PaCO2 in a healthy patient is 35–45 mmHg.
In an otherwise healthy patient who requires ventilation assistance under anesthesia, the target PaCO2 should therefore be in the normal physiologic range described above. There are a few clinical situations in which the risk of lung injury due to mechanical ventilation (to achieve normal PaCO2) outweighs the benefit of normal PaCO2. This ventilation approach is described as permissive hypercapnia.
The most common clinical scenarios in pediatrics that require a permissive hypercapnia ventilatory approach include most processes that involve lung hypoplasia. Premature infants, infants with congenital diaphragmatic hernia, and infants with primary or secondary lung hypoplasia fall into this category. Low tidal volume ventilation has been shown to improve important clinical outcomes in patients with such diseases, as they are particularly susceptible to permanent lung injury due to mechanical ventilation-induced volutrauma or barotrauma.
Other patient cohorts that may benefit from a low tidal volume ventilator strategy that results in permissive hypercapnia include those with acute respiratory distress syndrome. Finally, patients who have obstructive lung disease that does not allow complete exhalation, like severe asthma, may also benefit from permissive hypercapnia. Severe airway or bronchial obstruction increases the likelihood that inspiration will be initiated prior to the completion of expiration, creating intrinsic positive end-expiratory pressure (i.e., auto-PEEP). Ventilator strategies that allow complete exhalation, like decreasing tidal volume or inspiratory flow rate, necessarily may result in permissive hypercapnia.
The protective effects of hypercapnic acidosis may be a function of the acidosis or the hypercapnia per se, or a combination of both [34]. PaCO2 in the range 50–80 mmHg, with pH between 7.20 and 7.30, reflect typical levels observed with institution of this technique.
Hypocapnia
Hypocapnia can have potential significant health effects, specifically on cerebral perfusion. There are some clinical scenarios that can benefit from the physiologic changes induced by hypocapnia, but prolonged duration of hypocapnia can also have adverse neurologic consequences.
Mechanism of Neurologic Effects of Hypocapnia
Systemic hypocapnia results in cerebrospinal fluid (CSF) alkalosis, which in turn decreases cerebral blood flow through potent vasoconstriction of cerebral vessels [35,36]. This can lower intracranial pressure, and be life-saving in situations in which intracranial pressure is severely elevated [37,38]. Cerebral vasoconstriction also has potential negative effects. Excessive vasoconstriction can cause reduced oxygen supply to the brain, and alkalosis can lead to reduced oxygen release from hemoglobin, resulting in cerebral ischemia [39]. Hypocapnia may also lead to increased neuronal excitability [40]. Over time, with buffering of CSF pH, the cerebral blood flow may return to normal. This may result in relative cerebral hyperemia and reperfusion injury to previously ischemic brain regions.
Hypocapnia can be particularly injurious to the developing brain, especially in premature infants. Relatively less well-perfused areas of the premature brain can be particularly susceptible to the ischemia resulting from severe cerebral vasoconstriction caused by severe or prolonged hypocapnia [41]. This results in a specific type of white matter brain injury, such as periventricular leukomalacia. Changes in cerebral blood flow due to swings in PaCO2 can also result in intraventricular hemorrhage [42].
In summary, hypocapnia can have significant detrimental effects on cerebral perfusion, especially in premature infants. Close monitoring of ventilation, using blood gas measurements or noninvasive techniques, are critical to achieve optimal ventilation without negative pulmonary and neurodevelopmental effects.
References




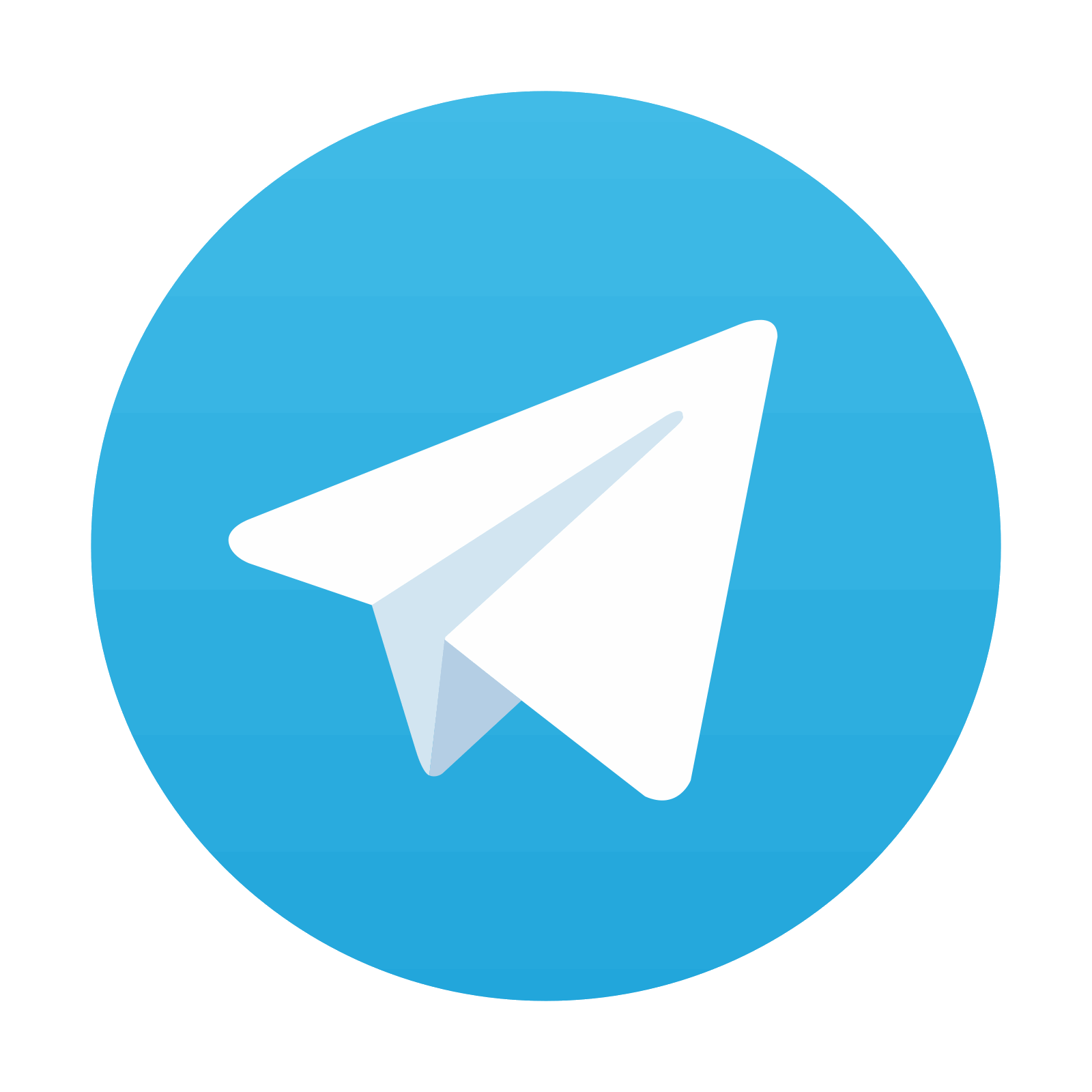
Stay updated, free articles. Join our Telegram channel
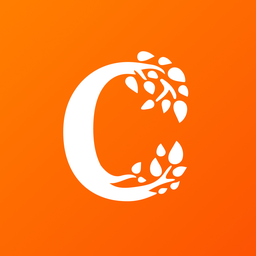
Full access? Get Clinical Tree
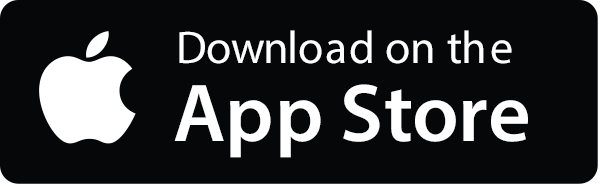
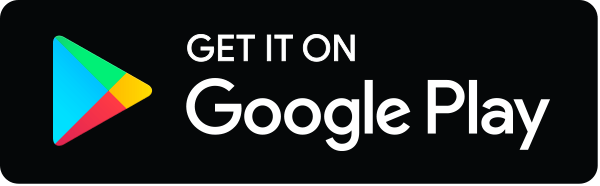
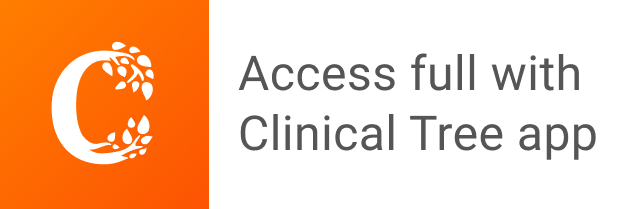