One of the key duties of the anesthetist, monitoring requires an advanced level of understanding of both how monitors work as well as how to interpret their data. An additional level of complexity is added when monitoring infants and children, as their physiology changes rapidly with age and may involve various abnormalities due to congenital disease.
Although monitors play a key role in the modern operating room, it cannot be overemphasized that keeping one’s eyes on the patient remains the most important method of detecting the evolution of critical events and evaluating the adequacy of the airway, breathing, and circulation. The goal of this chapter is to review commonly used and newly introduced monitors, the unique considerations of these monitors in pediatric patients, and how the information they provide can help detect critical events and guide management during anesthesia.
Central Nervous System Monitoring
A variety of monitors exist for the measurement of neurologic physiology and function. While these are most often used in cardiac and neurologic surgery, several are also becoming more common for use in general procedures as technology has improved in the area of noninvasive monitoring.
Intracranial Pressure Monitoring
Intracranial pressure (ICP) monitors, or ventricular catheters, are invasive monitors that sit inside the ventricle, where they can be used for continuous ICP monitoring, as well as drainage of cerebrospinal fluid (CSF) for therapeutic reduction of ICP. Infants often will not require them as open fontanelles allow for expansion of the intracranial contents without an increase in ICP, but they may be indicated in older children at risk for a pathologic increase in ICP. In general ICP is considered normal if less than 15 mmHg. It is typically 2–6 mmHg in term neonates and even lower in premature infants. Measuring both the mean arterial pressure (MAP) and ICP allows for calculation of cerebral perfusion pressure (CPP), which is defined as the difference between MAP and ICP. A high ICP decreases the CPP and can put the brain at risk for ischemia, thus monitoring of CPP is of utmost importance in patients with neurologic trauma or intracranial pathology. It has been suggested that children with traumatic brain injury should have their CPP maintained between 40 and 65 mmHg [1], a goal that can be guided by continuous pressure monitoring. As they are invasive monitors, ventricular catheters may cause hemorrhage or infection, and thus will typically be placed only in critically ill patients who may require intervention.
Electroencephalography
The electroencephalogram (EEG) utilizes a variable number of scalp electrodes to report electrical changes due to brain activity. Unprocessed EEG contains a wealth of information about neurologic function and can be used for monitoring in a variety of procedures, such as surgery on the carotid artery or epileptic foci, but requires specialized personnel for interpretation and is beyond the scope of most anesthesiologists. However, anesthesia produces characteristic changes in EEG function that give clues about the depth of anesthesia. Patterns such as burst suppression and electrical silence are easy to recognize as signs of an overanesthetized patient, while increased muscle activity in the non-relaxed patient suggests light anesthesia and impending movement.
Processed EEG, which includes proprietary monitoring systems such as the bispectral index (BIS®) or SEDline®, attempts to simplify unprocessed EEG into a more interpretable form. BIS monitors process EEG data using a propriety algorithm into a number from 0 (electrical silence) to 100 (full wakefulness) to report a level of consciousness [2]. BIS may be used for sedation monitoring and has been shown to correlate with clinical depth of sedation in children [3]. It also appears to correlate with depth of sevoflurane anesthesia in a manner similar to adults [4]. BIS can, however, be affected by developmental status – compared with neurodevelopmentally normal children, in children with cerebral palsy the same pattern of change is seen in response to anesthesia but overall BIS values are lower [5]. In infants BIS values are significantly lower for a given level of clinical sedation than in older children [6]. The use of BIS to prevent awareness during anesthesia has received considerable attention and remains controversial [7–9]. Although rates of awareness in children are similarly low to those in adults [10], no study has evaluated it for this purpose in pediatric patients.
Near-Infrared Spectroscopy
Using principles similar to those used for pulse oximetry, near-infrared spectroscopy (NIRS) utilizes near-infrared light to measure oxyhemoglobin and deoxyhemoglobin saturations in the cerebral vessels. The NIRS probe is placed on the scalp, where a light source emits two wavelengths of near-infrared light that traverse through the soft tissue of the scalp and the brain (Figure 19.1). The scattering and absorption of light is received by detectors where changes in hemoglobin saturation are analyzed and a saturation value known as the regional cerebral oxygen saturation index (rSO2i) is produced for both the right and left hemispheres. Although baseline readings are of limited value, an acute decrease in rSO2i suggests an imbalance between cerebral metabolism and blood flow. In general, a decrease in rSO2i of greater than 20 percent or an rSO2i reading of less than 50 percent is considered a threshold for intervention, with the goal of intervention being an increase in cerebral blood flow or a decrease in cerebral metabolic requirements depending on the most likely source of the disruption.
Figure 19.1 NIRS probe applied to the scalp. Near-infrared light of multiple wavelengths is emitted from a light source. The scalp and brain detector receive scattered and absorbed light from these tissues and output regional cerebral saturations.
Clinically, NIRS has been used in pediatric cardiac surgery, neurosurgery, and intensive care. A study of 20 pediatric cardiac surgical patients showed that rSO2i correlated well with mixed-venous oxygen saturation [11], suggesting it could be used as a marker for overall tissue oxygenation. In contrast to pulse oximetry, NIRS analyzes not just pulsatile blood flow but also nonpulsatile (i.e., venous) flow. Because of this, it can be used during states of nonpulsatile flow, such as cardiopulmonary bypass, and is of particular use in monitoring cerebral oxygenation during bypass and deep hypothermic circulatory arrest. Changes in NIRS signals were used in one case to diagnose aortic cannula malpositioning during cardiac surgery, which likely avoided a neurologic disaster [12]. A study looking at deep hypothermic circulatory arrest observed a smaller increase in cerebral oxygen saturation at the initiation of hypothermic cardiopulmonary bypass in patients who had postoperative neurologic changes [13].
Although NIRS is standard practice for pediatric cardiac surgery in many institutions, at this point the literature lacks a large study clearly demonstrating improved clinical outcomes with NIRS monitoring. This may be due to the fact that although NIRS can accurately identify a decrease in cerebral oxygenation, the provider must still diagnose and successfully treat the cause. NIRS sensors must be placed on the skin of the forehead and therefore hair, hematomas, or skull abnormalities may decrease the quality of signals. The requirement for forehead placement also makes NIRS unable to reliably detect changes in the posterior circulation.
Transcranial Doppler Sonography
Transcranial Doppler (TCD) signals monitor blood velocities to the brain via ultrasound signals through the skull. Typically probes are placed over the middle cerebral arteries where they can relay information regarding cerebral blood flow or embolic events, making TCD particularly popular for monitoring during cardiopulmonary bypass. The utility of TCD lies in its ability to detect changes in flow velocities [14], which can alert the user to acute changes in flow due to events such as hypotension or bypass cannula misplacement. However, the velocities themselves are poorly predictive of actual flow [15]. Like the precordial Doppler, TCD can detect embolic events and has been used during cardiac surgery in children [16].
TCD is limited by the need for operator skill to recognize changes in flow, and a lack of regular use reduces accuracy [17]. Signals can also be difficult to obtain in patients with thicker skull bones, although as infants and neonates have less-developed skulls they are among the easiest patients in which to obtain signals.
Evoked Potential Monitoring
Evoked potential (EP) monitoring is similar to EEG in that it uses electrical activity in the nervous system to monitor function. In contrast to EEG, which monitors spontaneous electrical activity in the brain, EPs result from artificial stimuli initiated at electrodes placed at specific peripheral monitoring sites throughout the body. An individual trained in EP monitoring typically places the leads and monitors nerve function periodically throughout the surgery to detect changes in neurologic function that may result from surgical manipulation. Important parameters of EPs include latency, defined as the length of time from stimulus to peak amplitude of the evoked response, and amplitude, which is the difference in voltages of the most positive and most negative peaks of deflection from the baseline reference potential.
The most commonly used forms of EPs in monitoring are brainstem auditory-evoked potentials (BAEPs), somatosensory-evoked potentials (SSEPs), and motor-evoked potentials (MEPs). BAEPs monitor the response to sounds and therefore can be affected by changes in much of the auditory pathway, including the cochlear, afferents of the vestibulocochlear nerve, and the brainstem. Brainstem auditory-evoked potentials are thus useful in neurosurgery and otorhinolaryngology, particularly in procedures around the skull base or involving the ear canal.
Somatosensory-evoked potentials are a monitor of peripheral afferent function. Recording takes place over the brain, and common stimulation sites include the ulnar, median, tibial, and common peroneal nerves. The technique is useful for monitoring for injury during complex spine procedures, such as scoliosis surgery, where its use has been demonstrated to decrease postoperative neurologic deficits [18]. Because their peripheral nerves are shorter, pediatric patients often have better signals than adults, and a decrease in amplitude of 50 percent or an increase in latency of 10 percent from baseline is defined as a change significant enough to warrant intervention.
Whereas SSEPs monitor afferent peripheral nerves, MEPs monitor efferent peripherals and can be combined with SSEPs for spine surgery to increase the sensitivity and predictive value versus SSEP alone [19]. MEP signals originate at electrodes over the scalp and motor potentials are elicited and recorded in the muscles of the hands and legs. A 50 percent decrease in amplitude is considered a significant change.
The use of EP monitoring requires several anesthetic considerations. Deepening an anesthetic can reduce the quality of EP signals, with MEPs affected the most and BAEPs the least. Volatile anesthetics have the largest effect on EP signals, thus these cases are performed using a total intravenous anesthetic when possible. MEPs also preclude the use of muscle relaxants and may be unreliable in patients with a preexisting neuromuscular deficit.
Neuromuscular Junction Monitoring
Because of their increased oxygen consumption and decreased respiratory reserve relative to adults, neuromuscular monitoring should be considered in all patients receiving nondepolarizing neuromuscular blockers (NDNMBs) when preparing for reversal or extubation. The major consideration in neonates and infants is that acetylcholine receptors are immature even at term, and continue to mature with age, which results in altered responses of infants and children to NDNMBs [20].
Neuromuscular blockade can be monitored by a variety of techniques. Qualitative clinical signs of strength, such as deep respirations, coughing, and thrashing, are good indicators of function. In infants, brow furrowing and hip flexion also are promising signs of activity, but clinical tests requiring language comprehension, such as a five-second head lift or hand squeeze, are impossible in infants and usually difficult in young children. As a result, it is prudent to use a twitch monitor before extubation of a patient who has recently received a NDNMB. Twitch monitors consist of two electrodes placed along a motor nerve connected to a source of current. The current is then applied between the electrodes along the nerve to stimulate acetylcholine release. Under ordinary circumstances this causes the innervated muscle to twitch, but if NDNMBs are present in the neuromuscular junction the twitch will be diminished or absent. The most popular locations to monitor include the ulnar nerve, facial nerve, posterior tibial nerve, and peroneal nerve, all of which are located close to the skin and innervate muscles that cause easily identifiable twitches. Larger children may have a large amount of subcutaneous fat which acts as an insulator and limits signal transmission to the nerve, sometimes to the point where a twitch cannot be elicited.
Twitches can be produced in a variety of patterns, most commonly the train-of-four (TOF). Current is applied a total of four times over two seconds (a frequency of 2 Hz) to produce four muscle twitches, and the ratio of the strength of the fourth twitch is compared to the first. A patient who has received a NDNMB will have a progressive decrease in the strength of each twitch until strength has returned. Zero twitches are ideal for intubation, while a ratio of 0.95 or greater is ideal for extubation. Premature babies and neonates have immature neuromuscular junctions and will often have decreased ratios at baseline in the first months of life, thus extubation criteria may need to be altered in this age group [21,22].
Other twitch patterns include the single twitch, tetany, and post-tetanic stimulation. The single twitch is less sensitive than the TOF at detecting residual blockade as it does not include further twitches to detect fade. Tetany applied at 50, 100, or 200 Hz will produce fade if blockade is present, but is very stimulating and not recommended if the patient is lightly anesthetized. A post-tetanic twitch is performed with a 5 s period of tetany followed by single twitches at 1 Hz. A patient who lacks a single twitch may have one after a period of tetany due to an increase in acetylcholine synthesis triggered by the tetany [23], making the post-tetanic twitch the earliest sign of the return of neuromuscular function. Like the TOF, post-tetanic stimulation is also less effective in infants with immature neuromuscular junctions.
Cardiovascular Monitoring
Anesthetists utilize similar cardiovascular monitors in neonates and infants as they use in adults. However, cardiac physiology and the vital signs change rapidly in the first year of life (Table 19.1), and an understanding of neonatal physiology is required for proper interpretation of commonly monitored parameters. In addition, the small size of infants makes placement of some invasive monitors difficult and their potential for congenital cardiac pathology requires flexibility in interpretation.
Age | Heart rate (beats min–1) | Blood pressure (mmHg) |
---|---|---|
Premature | 120–170 | 55–75/35–45 |
0–3 months | 100–150 | 65–85/45–55 |
3–6 months | 90–120 | 70–90/50–65 |
6–12 months | 80–120 | 80–100/55–65 |
1–3 years | 70–110 | 90–105/55–70 |
Precordial Stethoscope
Although less commonly used in the twenty-first century, the precordial stethoscope is an enormously useful monitor in pediatrics for detecting both cardiovascular and respiratory events. Typically the stethoscope is taped over the apex of the patient’s heart to free the hands of the anesthetist, and a fitted earpiece is placed in the anesthetist’s ear. Airway events such as apnea, bronchospasm, or obstruction, which are prevalent during mask induction, are detected more quickly than with the pulse oximeter or electrocardiogram (ECG). Changes in heart rate, blood pressure, or myocardial contractility can be detected as a change in the volume of heart sounds [25]. The precordial stethoscope does, however, require experience for proper interpretation and relies on sometimes subtle qualitative changes in volume that can be difficult to detect in the modern, noisy operating room.
Esophageal Stethoscope
The esophageal stethoscope is similar to the precordial stethoscope in practice, but sits in the esophagus instead of over the heart. It is obviously less useful for induction as placement requires an anesthetized, intubated patient. However, it is more convenient for intraoperative use. It detects the same events that a precordial stethoscope can, and recent work has shown it is also effective for detecting residual flow in patent ductus arteriosus closure [26]. As with any oropharyngeal invasive device, there is potential for trauma to the esophagus, airways, or displacement of the endotracheal tube.
Electrocardiography
The basis of ECG monitoring is the detection of electric potentials originating from the heart across various vectors on the chest. It is used continuously during anesthesia to detect a variety of changes in cardiac function that may result from anesthetic or surgical factors. In pediatrics the most common ECG changes are arrhythmias, thus patients are typically monitored with three leads that best display P-waves and QRS complexes. Ventricular leads, which are used to detect ischemia in adults, are often omitted due to the rarity of coronary artery pathology in pediatric patients. However, there are some conditions, such as Williams syndrome, that may involve coronary pathology and thus warrant the use of ventricular leads.
The arrhythmias most common in children are supraventricular tachycardia (SVT), atrial tachyarrhythmias, and sinus bradycardia [27]. Supraventricular tachycardia may be encountered during periods of high stimulation, such as intubation with light anesthesia. Atrial arrhythmias are seen in congenital heart disease with structural abnormalities. Sinus bradycardia is often the result of hypoxia and severe sinus bradycardia, also commonly seen on mask induction in children with Down syndrome. Halothane classically can cause various arrhythmias, but is no longer commonly used.
Indirect Arterial Pressure Measurement
Blood pressure (BP) can be monitored noninvasively via auscultation or oscillometry. Automatic oscillometric monitors are typically used during surgery and checked every 3–5 minutes. Oscillometric monitors measure BP by finding the pressure below which pulsatile flow returns in the artery, the systolic blood pressure (SBP), and the pressure at which the pulsatility in the artery has its highest amplitude, the MAP. Oscillometric monitors do not measure diastolic blood pressure (DBP) – it is instead calculated from the SBP and MAP and is frequently underestimated in children.
Noninvasive measurements are painless and easy in asleep and awake children, but can cause ischemic damage including nerve palsies and skin breakdown if repeated too frequently over the course of a long surgery. Cuff placement can affect the accuracy of noninvasive monitors. Placement on the distal arm or leg tends to overestimate SBP while underestimating DBP, although the MAP should be similar throughout the body in the absence of vascular disease. It has also long been known that a small cuff can overestimate the BP [28]. To avoid this, a cuff that is two-thirds to three-quarters in width of the upper arm length or, alternatively, a cuff that is wide enough to cover 40 percent of the upper arm circumference, should be chosen. Some cuffs come in sizes labeled “Infant,” “Neonate,” etc., but selecting a cuff merely based on age often yields an inappropriately sized cuff and therefore less accurate readings [29].
Direct Arterial Blood Pressure Measurement
For some procedures, or in the presence of some comorbidities where hemodynamics can change quickly, intermittent noninvasive blood pressure measurement may not be sufficient for safety. Other cases may require frequent blood sampling that would require multiple peripheral draws, which can be notoriously difficult in infants. In these cases placement of an indwelling arterial catheter is indicated.
Insertion of arterial catheters is described below and typically is performed with a 22- or 24-gauge intravenous catheter, although in older children and adults a 20-gauge is often used. The benefit of a larger catheter is a lower rate of clotting or kinking. After insertion, an arterial catheter is attached to pressurized, saline-filled tubing to ensure that retrograde flow, which can lead to exsanguination, does not occur. The tubing contains a transducer that is normalized to atmospheric pressure at the level of the aortic root or external auditory meatus, depending on whether the clinician wishes to monitor the pressures at the heart or brain, respectively. A monitor displays the arterial pressures transmitted through the tubing to the transducer. A typical arterial tracing in a normotensive patient has a brisk upstroke with a dicrotic notch, which diminishes the more peripheral the location of the arterial catheter. As a general rule, the further from the heart a catheter is placed, the higher the SBP will be and the lower the DBP will be, but the MAP should stay constant. A dampened tracing suggests catheter kinking, excessively long pressure tubing, or the presence of a clot on the tip of the tubing, but it is essential to assume any tracing or pressure reading is real until proven otherwise in order to expedite treatment in case a significant physiologic change has actually occurred.
Placement Techniques
Insertion of arterial catheters can be difficult in neonates. The artery is typically identified by one of three methods: palpation, ultrasound, or directly via surgical cut-down. Palpation is the most common method and is easiest in older children as their blood pressures increase and arteries enlarge, making the pulse easier to feel. One hand is used to locate the pulse while the other drives the catheter until a flash of blood is seen. The catheter can then be threaded in or can be placed with the Seldinger technique. For the Seldinger technique, the needle is often advanced through the back of the artery. The needle is then removed and the catheter pulled back until blood return is seen, confirming its placement in the lumen of the artery. At this point a wire is guided through the catheter into the lumen of the artery and the catheter is advanced over it into place. This method is especially useful in infants and neonates because they often do not have a palpable pulse. In the absence of a pulse, the needle is often advanced blindly and pulled back, repeated in different locations until blood return is finally obtained.
Insertion with ultrasound is a newer technique that is becoming more popular in infants due to their poor peripheral pulses. The ultrasound is first used to identify the targeted artery, either in a transverse or “out-of-plane” approach (Figure 19.2), or a longitudinal or “in-plane” approach. The needle is then advanced under ultrasound visualization as it enters the artery and is placed either with a wire or direct threading. Current literature on the efficacy of ultrasound-guided arterial line placement is mixed, with some studies on radial artery catheterization suggesting no improvement in the speed of placement [30] while others suggest that it improves speed, decreases the number of attempts required for placement, and decreases the rate of hematomas [31,32]. As practitioners become more facile with ultrasound technology it is likely that ultrasound placement will become more common, especially in neonates and infants.
Figure 19.2 Surgical cut-down and ultrasound approaches to radial artery catheterization. The cut-down utilizes direct visualization after dissection to the level of the radial artery. Ultrasound imaging can be used to locate the artery and the IV catheter observed to enter the vessel in a two-dimensional slice.
Surgical cut-down, the process of dissecting tissue down until the artery can be directly visualized and cannulated, is effective as both a first-line placement as well as when other methods have failed (Figure 19.2). Although it is the standard method for placement in small infants at many institutions, it has been associated with increased rates of complications, which will be discussed in a later section.
Cannulation Locations
Arterial lines can be placed in a variety of peripheral arteries, the most popular of which is the radial. The technique is performed at the radial aspect of the wrist, where the radial artery is straight, superficial, and palpably pulsatile. Radial artery cannulation is considered safe because of collateral flow to the hand from the ulnar artery – limb ischemia is extremely rare, but it is nonetheless advisable to check for collateral flow by observing perfusion with compression of the radial artery (Allen’s test) prior to attempting placement. Radial arteries and all other upper extremity arterial lines may have different readings on the right and left sides in infants with pathology involving the aortic arch, such as coarctation of the aorta. In such conditions the right radial artery is preferred as the brachiocephalic trunk leaves the aortic arch the earliest and has the highest likelihood of branching before the lesion. Surgically altered anatomy, such as a Blalock–Taussig shunt, may also affect pressures depending on the site and should be considered accordingly. Some congenital syndromes, such as Down syndrome, are also associated with abnormalities in the radial arteries. One study of Down syndrome patients found one or more abnormalities in 16 percent of patients’ forearm arterial trees [33].
An alternative to the radial artery in the upper extremity is the brachial artery, which is larger and often has a better pulse than the radial [34]. Although considered safe, it is important to remember that the brachial is an end-artery and occlusion will stop blood flow to the entire extremity in the absence of collateral circulation. The artery is found at the medial aspect of the antecubital fossa and is easiest to cannulate there.
Other commonly chosen arteries are the temporal, femoral, posterior tibial, and dorsalis pedis. The temporal artery can be found at the temple and is occasionally used in neonates, but is difficult to place well and is easily displaced by a moving child. The femoral artery is found just distal to the inguinal ligament between the pubic tubercle and anterior superior iliac spine. As it is the largest peripheral artery it is the easiest to cannulate, making it a popular choice in small neonates and infants or in emergent situations. The posterior tibial is found posterior to the medial malleolus and the dorsalis pedis is found on the dorsum of the foot. As they are the farthest point in the body from the heart, both arteries will have grossly overestimated SBPs and underestimated DBPs. Placement should never be attempted in both a dorsalis pedis and posterior tibial artery on the same limb as the arteries form a collateral network and blocking both would cut all circulation to the foot.
A unique option for catheterization in the neonate is the umbilical artery, which compared to other artery choices offers both major benefits and potentially devastating complications. As it is used in neonates that are usually in an intensive care setting who may need months of inpatient treatment, the umbilical artery is often used first as it spares other sites that may be needed for catheterization in the future. It is the only artery that also can be a route for medication administration. Umbilical catheters are placed by identifying and puncturing one of the two umbilical arteries on the umbilical cord and advancing a catheter into the descending aorta. As the neonate is very small, even a slight movement of the catheter can result in occlusion of major vessels and ischemia. A short list of things that can be occluded by umbilical catheters includes the iliac, femoral, and brachial arteries, chambers of the heart itself, and, if it were to traverse a patent ductus arteriosus, the pulmonary artery. Catheters can also cause infection, embolic events, vascular puncture and hemorrhage, and arrhythmias [35].
Risk/Safety Profile of Arterial Lines
In general, arterial lines are very safe and have a low rate of infection – lowest if inserted with sterile technique [36,37]. As it is highly invasive, the cut-down technique is associated with a higher rate of infection [38].
A concern in children who will undergo multiple arterial cannulations, such as those with congenital heart disease, is occlusion and eventual scarring of the artery. Pediatric patients have higher rates of arterial occlusion after catheter placement; however, this typically resolves several days after removal. Placement by the cut-down technique or prolonged catheterization makes occlusion less likely to resolve [39]. Hematoma formation is another threat to arterial flow that may be reduced with fewer arterial punctures and, potentially, the use of ultrasound for placement [31]. Blood loss must also not be forgotten during placement and subsequent blood draws, especially in neonates and small infants with low blood volumes. Losses during sampling draws can be decreased by using shorter lengths of tubing between the artery and the stopcock. Blood loss can also be a result of low pressure in the tubing due to a disconnection from the pressure bag or a lack of pressure in the bag, with either scenario having the potential to cause life-threatening hemorrhage.
Central Venous Access and Central Venous Pressure
Obtaining central venous access is indicated when there is a lack of adequate peripheral access, the need for vasopressors or other medications that are hazardous when administered peripherally, measurement of central venous pressure (CVP), and, during high-risk cases, aspiration of air emboli from the heart. Central lines are placed most often in the internal jugular, femoral, or subclavian veins, but can also be inserted into the external jugular or basilar veins in some cases. In neonates the umbilical vein can be accessed in a similar method as the umbilical artery. Umbilical vein catheters have many of the same hazards as their arterial counterparts, most notably potential migration and subsequent venous obstruction in the liver.
Placement of Central Venous Catheters
The techniques for locating large veins in infants are similar to those used in adults. The basilar, external jugular, and umbilical veins can be directly visualized for puncture. The palpation and landmark techniques used for subclavian, femoral, and internal jugular lines in adults can be used in the same manner in pediatric patients, although it should be noted that the internal jugular vein is often located more cephalad in infants than in older children and adults. Ultrasound-guided line placement was widely used in infants before becoming routine in adults, can be used to locate any of the commonly cannulated large veins, and has been demonstrated to improve success rates, reduce carotid artery punctures, and increase the speed of placement [40,41].
Regardless of how the vein is located, the Seldinger technique is typically used to place the line. A needle attached to a syringe with negative pressure applied by the inserter is advanced until blood is aspirated into the syringe, confirming placement in the vein. The syringe is then removed and a wire advanced into the vein so the needle can be removed; during this stage it is essential that the ECG be monitored as the wire can cause arrhythmias if it is advanced too far and comes into contact with the walls of the heart. After widening the opening in the skin with a skin blade, a dilator is inserted over the wire and then removed to clear a path for the catheter. The catheter is then advanced into place and the wire removed. Catheters placed in the basilar, external jugular, internal jugular, and subclavian veins may have distal tips capable of reaching the heart. Care must be taken to make sure the tip of the catheter does not sit within the heart – to accomplish this, central venous catheters that are going to be used long-term are often placed in the operating room under fluoroscopy. If the clinician wishes to monitor CVP, the catheter should terminate at the junction between the superior vena cava and the right atrium.
The complications of central lines are the same as those in adults. Infection is a common complication and unless placed in an emergency all central venous lines should be inserted using sterile preparation, draping, and technique. Catheter migration into the heart may cause arrhythmia and all catheters should have placement confirmed radiographically. Pneumothorax is a concern for internal jugular and subclavian lines. Bleeding can also be significant if CVP is high or placement is prolonged. Inferior vena cava thrombosis was detected in six of 56 children with femoral lines in one study [42]. All of these children had their catheters for more than six days, and it is thus recommended that catheters be monitored for thrombosis or changed after six days.
Measurement of CVP
A properly placed central line can be used to obtain CVP, the pressure recorded at the junction of the right atrium and superior vena cava. Continuous monitoring of pressure in the central vein creates a tracing with three ascents: the a-wave, which is caused by atrial contraction; the c-wave, which is caused by ventricular contraction and upward tricuspid displacement; and the v-wave, which is the result of rapid venous filling against a closed tricuspid valve. The number reported as the CVP is the value obtained at end-expiration and has long been used as a surrogate marker for volume status. Recent work in adults [43] has called into question whether CVP correlates with volume status, although many still use it in conjunction with other data to assess volume status.
Pulmonary Artery Catheters
Although infrequently placed in pediatric patients, pulmonary artery catheters can provide useful measurement of cardiac output, mixed venous oxygen saturation, and pressures in the right atrium, right ventricle, and pulmonary artery. This information can be useful, especially in the setting of abnormal cardiac physiology, particularly in cardiac surgical patients with severe pulmonary hypertension or anatomical defects [44]. However, interpretation in these children requires a flexible interpretation of normal values as significant shunting or anatomical abnormalities can result in major changes in readings at baseline.
Pulmonary artery catheters are placed through central venous catheters, typically an internal jugular, subclavian, or femoral line. The size of infants makes placement extremely difficult and is fraught with complications including infection, arrhythmia, pulmonary artery rupture, and bleeding. A recent review [45] showed that although there was no increase in mortality from pulmonary artery catheter placement, there was also little evidence in favor of placement in pediatric patients.
Transesophageal Echocardiography
Transesophageal echocardiography (TEE) is an increasingly utilized method for monitoring cardiac function during both cardiac and noncardiac surgery. Its efficacy has been demonstrated in cardiac surgery for diagnosing intraoperative problems and guiding medical and surgical therapy [46]. Transesophageal echocardiography must be used cautiously in infants and neonates because the heavy probe can cause compression of vascular and respiratory structures, as well as esophageal perforation and vagal responses. As a result, its use is limited to situations where TEE information may change the course of a procedure or provide vital diagnostic or physiologic information.
Respiratory Monitoring
Neonates and infants have multiple anatomic and physiologic features that make them prone to perioperative respiratory events. At birth a neonate has a rate of oxygen consumption twice that of an adult, a smaller functional residual capacity while anesthetized, and a variety of airway features that leave little room for error during anesthesia. After anesthesia, many remain at a high risk for respiratory events including apnea of prematurity [47]. A strong understanding and awareness of respiratory monitors is therefore essential for pediatric anesthesiologists.
Pulse Oximetry
The pulse oximeter determines oxygen saturation via a probe placed on a peripheral location, often a finger or toe, sometimes the earlobe or forehead. The probe emits light at two specific wavelengths, which are absorbed differently by oxygenated and deoxygenated blood, allowing the oximeter to determine the percentage of hemoglobin that is oxygenated via an algorithm. The pulse oximeter also uses plethysmography to determine pulsatile flow and report saturation information during systolic beats. In this way, the pulse oximeter is also a sensitive monitor of peripheral perfusion.
The pulse oximeter has been used in children since early in its clinical life. One of the first studies on the effectiveness of the pulse oximeter was performed on children and showed that practitioners blinded to pulse oximetry data had more hypoxic events and were slower to identify hypoxic events than those who had the data available, although there was no difference in overall morbidity [48]. A follow-up study that added capnography [49] identified the pulse oximeter as the best monitor for identifying desaturation events and a serviceable one for detecting events typically identified by capnography, such as esophageal intubation or endotracheal tube kinking, although capnography and pulse oximetry together showed the best overall results.
Several considerations are relevant for accurate pulse oximetry use in pediatrics. The pulse oximeter is disrupted by any kind of motion [50], making it somewhat impractical in awake children such as those being induced via mask. Skin color and thickness minimally affect accuracy. Environmental factors can affect pulse oximeter signals. Electrocautery and some types of lighting can interfere with readings [50]. Nail polish, especially blue and green in color, can artificially decrease oximeter readings [51]. Stereotactic guidance systems, often used for neurosurgical procedures, use infrared light and disrupt pulse oximeter signals [52], although this can be averted by placing an opaque wrapping over a probed finger. If there is concern for inaccurate readings during a procedure, two pulse oximeters are often used in different locations.
Pigments within the bloodstream also affect oximeter signals based on their light absorption spectra. Intravenous dyes, such as methylene blue, indigo carmine, and indocyanine green can all falsely decrease readings [50]. Abnormal hemoglobins and related pigments may also alter readings. Fetal hemoglobin, carboxyhemoglobin, and bilirubin do not affect signals, but the presence of methemoglobin will decrease readings, classically to 85 percent, regardless of the actual saturation [50]. If methemoglobin or carboxyhemoglobin levels are suspected to be high they can be detected by multiwave pulse oximeters (CO-oximeters) that use extra wavelengths of light to identify different forms of hemoglobin [53,54].
Placement of pulse oximeters requires special attention in infants with congenital heart disease. Pulse oximeters are designed to be most accurate at higher saturations and tend to lose accuracy at lower ones, especially saturations lower than 80 percent [55], which may limit their use in children with cyanotic lesions or lung disease. Patients with patent ductus arteriosus may have a variety of readings depending on where the probe is placed. A preductal probe on the hand or ear will report preductal saturations and is useful for monitoring cerebral oxygenation. A postductal probe placed on the foot will report postductal saturations, which is especially important in conditions with significant right-to-left shunting, such as persistent pulmonary hypertension of the newborn. In practice, however, a difference in saturations is not always seen, as the ductus arteriosus often attaches distal to where the left subclavian artery leaves the aorta. A study of newborns revealed no significant decrease in left-hand pulse oximeter saturations during the first four hours of life [56].
Capnography
By continuously sampling the CO2 levels near the endotracheal tube and graphing the levels in real time, the CO2 analyzer creates an accurate graphical representation of the patient’s ventilation, known as the capnograph. Carbon dioxide analyzers use infrared light to measure the CO2 content in the expired gas. The sample can be taken from either a sidestream analyzer, which removes gas from the circuit and analyzes in a separate unit, or via a mainstream analyzer that detects gas in the circuit via a cuvette that is inserted into the tubing. Mainstream analyzers are less prone to obstruction but require cuvette sterilization and replacement. Although they rely on small tubing that can be obstructed with condensation or secretions, sidestream analyzers are the most common in practice today.
Capnography is used in anesthesia to monitor gas exchange during anesthesia with or without an endotracheal tube. When used to guide mechanical ventilation settings, continuous capnography has been shown to decrease intraoperative hyper- and hypoventilation in children [49]. The monitor is also accurate for monitoring respiration during sedation via long tubing with nasal cannula or facemask when the patient may not be immediately accessible, such as during MRI procedures [57]. Capnography is also used to diagnose events manifested by altered end-tidal CO2 readings, such as esophageal intubation, hypoventilation, circulatory arrest, pulmonary embolism, or bronchospasm [58,59]. Hypercarbia is among the most sensitive signs for malignant hyperthermia and the capnograph can be used to both diagnose and guide treatment [60]. Endotracheal tube kinking or disconnection is also easily detected [61].
Capnography can be difficult in children due to their size and respiratory physiology, both of which contribute to widening of the alveolar CO2 (PACO2) to end-tidal CO2 (ETCO2) gradient. Sidestream analyzers typically aspirate 50 ml min–1, and fresh gas can be entrained if tidal volumes are low and the aspiration rate nears the expiratory flow rate – thus smaller children tend to have higher PACO2 to ETCO2 gradients [62]. Small tidal volumes also mean that to ensure minimal mixing with fresh gas, the sampling should be done as close to the patient as possible. The shape of the capnograph is often rounded at the peaks and troughs in pediatric patients, which is an artifact that can be caused by high respiratory rate or leak around an uncuffed tube. Cardiopulmonary pathology can also result in underreported CO2 levels, such as in the presence of a left-to-right shunt or severely decreased cardiac output.
Inhaled and End-Tidal Gas Monitoring
In most modern anesthesia machines, the sidestream aspirator used to deliver CO2 for capnography is also used to deliver gases for analysis of inhaled anesthetics, nitrous oxide, and oxygen by infrared spectroscopy. Monitoring of oxygen allows for avoidance of delivery of a hypoxic gas mixture while monitoring of inhaled agents helps prevent over- or underanesthetizing the patient. Although the monitor may reliably report the concentration of inhaled anesthetic, this information should not be mistaken for a measurement of the depth of anesthesia, which requires an assimilation of information from physical examination and various monitors by the anesthesiologist. Pediatric patients come for surgery at many stages of development and the level of anesthetic needed for each will vary.
Older anesthesia machines may use mass spectroscopy to measure end-tidal gases, which allows for the measurement of end-tidal nitrogen (ETN2). End-tidal nitrogen can be used to determine when nitrogen washout has been satisfactorily completed during preoxygenation, and was shown to detect air emboli more quickly than ETCO2 in a canine model [63]. As modern anesthesia machines almost exclusively use infrared spectroscopy for gas analysis, ETN2 is rarely used in today’s practice.
Temperature Monitoring
Neonates and infants are at a high risk for intraoperative hypothermia; a full discussion of temperature regulation is found in Chapter 8. The induction of general anesthesia relaxes the body’s tight control of core temperature, making patients vulnerable to various forms of heat loss in the operating room: radiant heat loss to the cold operating room; conductive loss from cold intravenous fluids or blood products; evaporative heat loss from exposed skin or viscera or from the airways during mechanical ventilation; and convective heat loss to the air or breathing circuit [64]. Conversely, hyperthermia can also be a threat due to aggressive warming techniques or malignant hyperthermia. Because pediatric patients have a high surface area to volume ratio and often have less fat tissue for insulation, temperature monitoring and modulation with appropriate warming or cooling mechanisms is standard for most procedures and essential for those involving tight temperature control, such as procedures requiring cardiopulmonary bypass.
The most common thermometers used in operating rooms are disposable thermistors or thermocouples. A thermistor contains a semiconductor that changes resistance with a change in temperature. A thermocouple is a circuit made of two different metals as electrodes. One of the electrodes is kept at a fixed temperature while the other is placed distally on the probe and used to measure the patient’s temperature. The difference in temperatures creates a gradient in the circuit that results in the flow of current, with the degree of flow used to determine the temperature on the probe. Thermistors and thermocouples can be used for monitoring in essentially any location. Skin temperature can also be monitored by liquid crystal thermometry. These thermometers are typically strips placed on the forehead that change color when a specific temperature is reached, but they are typically less accurate and should be reserved for simple procedures where tight temperature control is not essential [65].
The location to monitor temperature is based on accuracy, safety, ease of placement, and operative considerations. For most procedures the goal of temperature monitoring is to reflect the core temperature, which is the temperature of central tissues that receive the majority of the blood flow. Peripheral tissues are often cooler than the core compartment and in general the more peripheral the site, the greater the discrepancy with core temperature due to environmental factors [66].
Nasopharyngeal monitoring is considered safe in the absence of anatomic defects in the region (e.g., basilar skull fracture) and accurately reflects core temperature when placed in contact with the soft palate. It is ideal for monitoring of brain temperature during cardiopulmonary bypass. Nasopharyngeal monitors can lose accuracy if they are not placed in contact with the soft palate, are placed too high in the oropharynx, or come into contact with cold respiratory gases, such as when an uncuffed tube with a significant leak is placed.
Esophageal probes also accurately reflect core temperature and are easy to place. They can be combined with an esophageal stethoscope as a single piece of equipment to insert. Ideally they should be placed in the distal esophagus where the heart sounds are loudest. In small children, esophageal probes can come into close contact with the airways, which can skew the measurement based on inspired air temperature [67]. It may also be less accurate during cardiac or thoracic procedures with an open chest.
The axilla is a convenient, noninvasive location for temperature monitoring that can accurately reflect core temperature if placed correctly [67]. It is essential that the probe is in tight contact with the skin for an accurate reading. Axillary probes come into contact with several mechanisms that can reduce their accuracy, such as intravenous fluids being infused through the ipsilateral arm or external warming blankets.
The skin is an easy, noninvasive monitoring location that is considered less accurate than the others mentioned, especially as the sensor is placed in more peripheral locations [67]. A recent study suggested that measuring skin temperature over the carotid artery accurately reflected core temperature provided that a correction factor of 0.52 °C was added to the reading [68].
Rectal temperature is considered accurate for procedures that do not involve heating or cooling of the abdomen or pelvis. Accuracy can also be lowered by large amounts of stool in the rectum which reduce contact with the mucosa. Rectal probes cannot be placed in patients with an imperforate anus and should be avoided in patients at risk for serious damage should trauma occur to the rectum, such as those with friable tissues (e.g., inflammatory bowel disease) or coagulopathy. Rectal temperatures also lag esophageal and nasopharyngeal measurements in active cooling and warming during cardiopulmonary bypass procedures [69].
Bladder temperature can be measured via a probe inserted as part of a Foley catheter. It is accurate in most conditions but, similar to rectal monitoring, it lags other locations during rapid changes in cardiopulmonary bypass procedures [69].
Pulmonary artery catheters provide very accurate measurements of core temperature, but as pulmonary artery catheters are infrequently placed and involve considerable risks of perforation or arrhythmia, they are uncommonly used. During cardiopulmonary bypass the catheter is isolated from circulation and unusable.
The tympanic membrane is traditionally used as the gold standard for temperature monitoring as it approximates the temperature of the hypothalamus [70], although it has not been shown that hypothalamic temperature reflects core temperature. The probe is inserted into the external auditory canal but should not contact the tympanic membrane itself, as it can cause damage to the delicate structures within the ear. This location is used infrequently for continuous monitoring as studies have demonstrated that other sites can accurately reflect core temperature with easier placement and less risk [66,67].
References

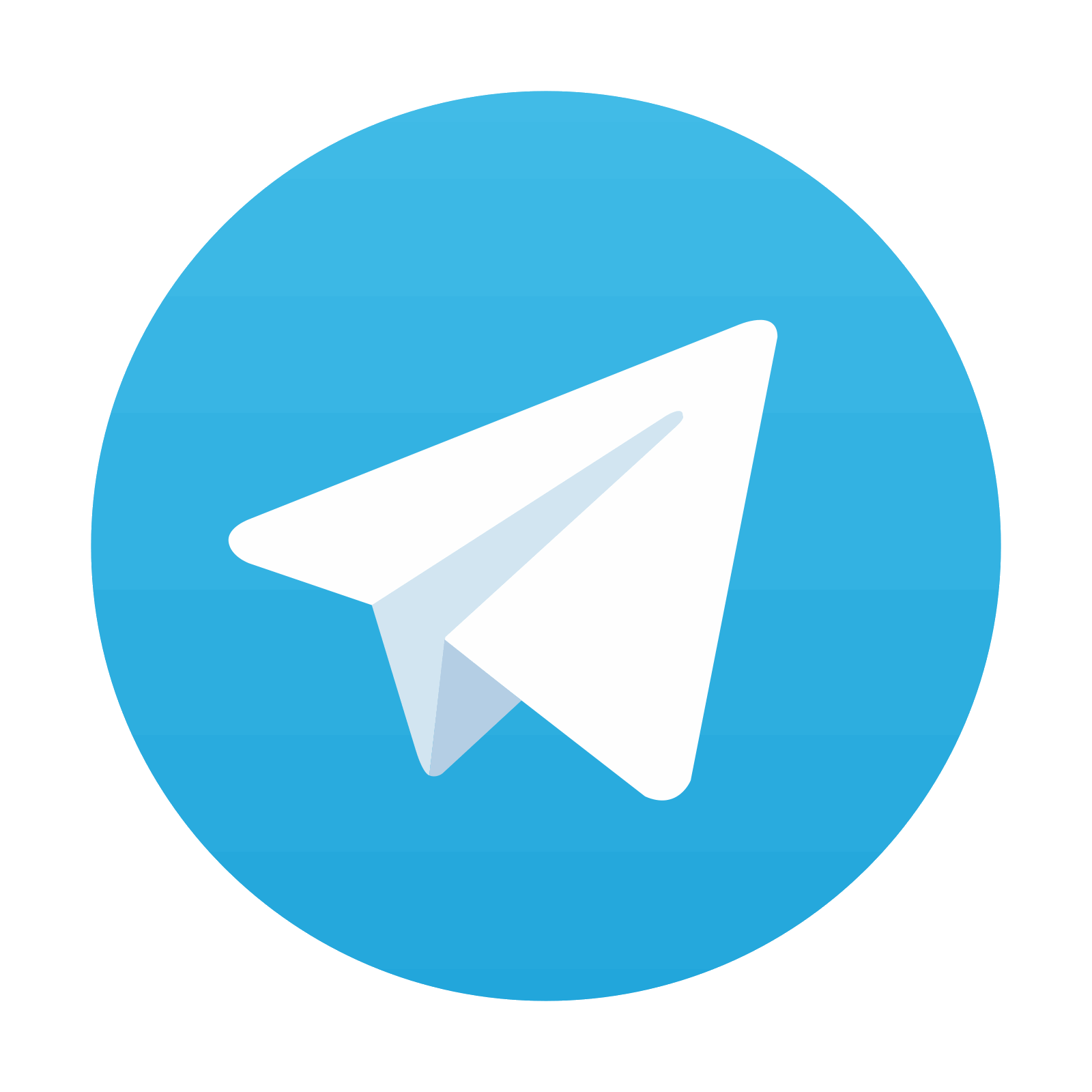
Stay updated, free articles. Join our Telegram channel
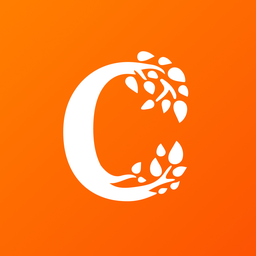
Full access? Get Clinical Tree
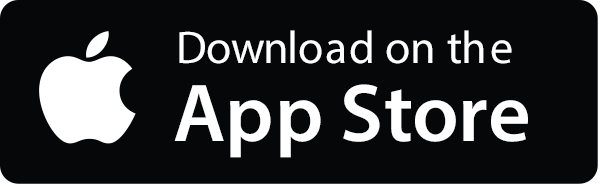
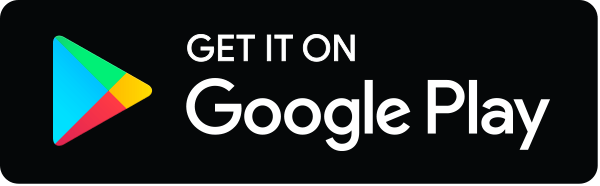
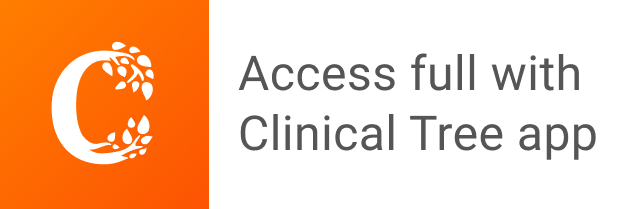