Introduction
Neonatal lung injury differs from acute lung injury (ALI) in older children and adults in a number of ways. Neonatal respiratory failure is the endpoint of several disease pathways. For instance, respiratory failure in the preterm infant is associated primarily with surfactant deficiency and is lacking the inflammatory and infectious properties of ALI in older patients. Persistent pulmonary hypertension (PPHN) of the newborn is caused by pulmonary artery hypertension, and in PPHN physiology, fetal circulation (systemic pulmonary artery pressures and a persistent ductus arteriosus with shunting) usually persists in the setting of acidosis, hypoxemia, infection, and thermal stress of the neonate. Meconium aspiration syndrome is associated with surfactant inactivation and lung inflammation.
In contrast to adult respiratory failure, nonconventional modes of ventilation such as high-frequency ventilation (HFV), the use of inhaled nitric oxide (iNO), and extracorporeal membrane oxygenation (ECMO) have a long tradition in neonatal ventilation, are used more liberally in neonates compared to the adult clinical arena, and are associated with improved outcomes in selected neonatal patient populations.
Monitoring and Goals of Gas Exchange
Pulse oximetry is commonly used to monitor oxygenation in neonates. However, SpO2 is an unreliable tool with regards to detecting hyperoxia. If SpO2 is above 95–96 percent, the corresponding PaO2 may be above 90–100 mmHg, raising concerns for increased oxygen toxicity in neonates. The ideal transcutaneous saturation in neonates during mechanical ventilation should probably be around 90–95 percent. In the SUPPORT trial, a transcutaneous saturation of 85–89 percent was associated with increased mortality compared to a saturation of 91–95 percent, with no difference in the rate of severe retinopathy [1]. In neonates with pulmonary hypertension and large right-to-left shunts, such as patients with PPHN or congenital diaphragmatic hernia, a difference in pre- and postductal saturations is observed. The preductal saturation is usually measured with an arterial line or probe on the right arm. The postductal saturation is usually measured by a left-arm arterial line, a lower extremity arterial line, or an umbilical artery catheter, as the tip of the umbilical artery catheter is usually in the aorta, reflecting a postductal saturation. In cases with significant shunting, treatment is usually adapted to target a preductal saturation of 85–95 percent and a postductal saturation above 70 percent.
Ventilation is noninvasively monitored by end-tidal carbon dioxide or transcutaneous carbon dioxide sensors. The presence of end-tidal CO2 is a function of pulmonary blood flow. Increased amounts of pulmonary dead space usually accounts for a large gap between arterial and end-tidal carbon dioxide measurements. If pulmonary dead space increases, the end-tidal CO2 is considerably lower than the arterial CO2. Low cardiac output, low pulmonary blood flow (such as in pulmonary hypertension), and airway obstruction can also lead to low or even absent end-tidal CO2 levels, despite correct tracheal position of the endotracheal tube. Regarding goals for CO2 levels, some degree of permissive hypercapnia is an accepted strategy, indicated by a pH above 7.25 and a CO2 level less than 60 mmHg. Hyperventilation, on the other hand, may lead to an alkalosis, resulting in cerebral vasoconstriction, low cerebral blood flow, and the potential for intracerebral hemorrhage.
Setting Up Ventilation Parameters for Neonates
Conventional Ventilation
Limiting delivered tidal volumes and optimizing alveolar recruitment, thus minimizing atelectrauma [2] and volutrauma [3], is one of the main strategies of lung protective ventilation in neonates with acute lung injury. Most centers attempt to limit peak inspiratory pressures to 25 cmH2O, and start with a peak inspiratory pressure of 15–20 cmH2O and a PEEP level of 3–5 cmH2O. Respiratory rate is usually adjusted to achieve PaCO2 levels of 45–60 mmHg. During conventional ventilation, tidal volumes are usually targeted to be 6 ml kg–1 body weight, with a level of positive end-expiratory pressure that allows the FiO2 to be weaned under 0.6 [3]. Lower tidal volumes have shown to be lung protective compared to larger tidal volumes in neonatal and adult patients. The inspiratory time is usually set at 0.3–0.4 seconds. During pressure-controlled ventilation with a decelerating inspiratory flow, the decelerating flow at the end of inspiration can be used to determine the ideal inspiratory time: the flow at the end of inspiration should reach the baseline. A noncompliant lung usually requires a shorter inspiratory time (0.2–0.3 seconds), a compliant lung a longer inspiratory time (0.4 seconds). In premature neonates, this effect can be observed before and after application of exogenous surfactant: prior to surfactant, the lung has a lower compliance, requiring a shorter inspiratory time; after surfactant, lung compliance increases, resulting in a longer inspiratory time during pressure-controlled ventilation with decelerating inspiratory flow.
Persistent hypercapnia and respiratory acidosis in neonates in the absence of obstructive physiology is often associated with obstructed endotracheal tubes. The inner diameter of neonatal endotracheal tubes may be as small as 2.0–2.5 mm. Often, suctioning the endotracheal tube does not improve the hypercapnia and the suction catheter may pass easily through the endotracheal tube. However, in this circumstance, changing out the endotracheal tube may be warranted. Figure 16.1 shows a 2.5 mm endotracheal tube after removal with large amounts of mucous narrowing the internal orifice. This infant had suffered from persistent respiratory acidosis, and was weaned to extubation shortly after endotracheal tube change.
Figure 16.1 Obstructed ETT (2.5 mm) after removal. This premature baby had persistent respiratory acidosis and hypercapnia. Expiratory flow curves and end-tidal CO2 tracing showed no characteristic signs of obstruction. Suctioning of the endotracheal tube was possible without problems. Regardless, the ETT showed severe obstruction by mucous and debris. The infant was extubated within 24 h after changing the ETT.
High-Frequency Ventilation
High-frequency ventilation allows sustained recruitment of atelectatic lung units while delivering small tidal volumes. High-frequency ventilation can be delivered through slightly different options. High-frequency oscillatory ventilation (HFOV), high-frequency jet ventilation, and high-frequency flow interruption all have in common that small (1–3 ml kg–1) tidal volumes approximating the anatomical dead space are delivered at rates exceeding the normal respiratory rate (3–20 Hz, 180–1200 breaths per minute). Data from a neonatal trial indicated a small benefit of HFOV in terms of pulmonary outcome for very low birth weight infants. Although no improvement in mortality or ventilator-free days during HFOV has been shown, there is a large body of evidence that HFOV is a safe strategy of ventilation allowing rapid and effective recruitment of lung volume [4].
Mechanisms of Gas Exchange During HFOV
While HFOV is frequently used in the neonatal intensive care unit, it appears physiologically counterintuitive that this modality of ventilation produces both adequate oxygenation and removal of CO2. The physiology of HFV is sometimes explained with the spontaneous respiratory physiology of panting dogs, who are capable of respiratory rates of 5–6 Hz (240–300 breaths per minute) while they are panting. Adequate gas exchange in dogs is maintained despite small tidal volumes during rapid breathing [5]. However, this model of comparison is lacking the important fact that during spontaneous respiration, a negative inspiratory pressure is generated, whereas during HFV, positive pressure is applied. In reality, during HFOV, gas exchange is achieved by a number of different mechanisms, which all interact with each other in the neonatal lung. The various modes of gas exchange are different in the larger airways, the proximal alveoli, and on the alveolar level (Figure 16.2).
In the proximal lung units with short path lengths, direct ventilation of alveoli by bulk flow is predominant. Even small tidal volumes reach the most proximal alveolar units directly, resulting in direct ventilation of this fraction of lung units. This mode of gas exchange closely resembles conventional ventilation, and, to use the earlier example, is probably the predominant mode of ventilation in panting dogs.
In large airways such as the trachea and the left and right main stem bronchus, Taylor-type dispersion and convective dispersion occur. Taylor, in 1953, described the dispersion of particles in the presence of laminar flow [7]. When the velocity of gas flow increases, the initial planar surface of a gas column transforms into a parabolic surface, allowing a greater amount of longitudinal mixing and dispersion. The center of the parabolic gas column is believed to travel faster than the outer areas, allowing further diffusion and mixing downstream. Turbulence occurring when this gas column reaches a bifurcation partly replaces laminar mixing, resulting in dispersion of gas molecules, further contributing to gas exchange. Convective dispersion occurs when a uniform column of air is transformed into a parabolic shape. Air molecules undergo mixing as molecules in the center of the gas column move to the tip of the parabolic shape and the molecules near the wall stay behind.
In the peripheral airways and the alveoli, Pendelluft, molecular diffusion, and cardiogenic mixing play the main role. Pendelluft (from German: pendel = pendulum and luft = air) means that gas moves between compliant and noncompliant lung units, resulting in regional mixing. Pendelluft is believed to occur during both inspiration and expiration. At end-expiration, air moves from the compliant to the noncompliant lung unit, as the compliant unit has a higher time constant and is still emptying when the noncompliant area is already empty. At end inspiration, air moves from the noncompliant area, which secondary to a lower time constant is filled earlier, to the compliant area, which is still filling [8]. Passive diffusion of gas molecules through the alveolo-capillary membrane (molecular diffusion) and cardiac-induced pressure changes in the vascular bed (cardiogenic mixing) both add to gas mixing on the alveolar level. Both mechanisms of gas exchange occur during any form of ventilation and are not unique to HFOV [4].
Setting Up and Maintaining HFOV in Neonates
Indication and General Considerations
High-frequency ventilation is considered in the setting of failing conventional ventilation in patients with acute lung injury. In addition, HFV is considered when potentially lung-injurious ventilator settings are applied. This is usually indicated by arterial hypoxemia despite peak inspiratory pressures of >25 cmH2O or oxygenation indices (FiO2 × mean airway pressure × 100/ PaO2) of >13 on two or more arterial blood gas analyses within a six-hour time period. Unlike older children or adults, neonates can breathe spontaneously during HFV, as the bias flow of the system is sufficiently high to support spontaneous ventilation in neonatal, but not pediatric or adult patients.
Considerations for Exclusion
Diseases with increased airway resistance, as seen in bronchiolitis and reactive airway disease, are generally associated with decreased ventilation, hypercarbia, and air trapping. The application of an aggressive recruitment strategy may increase the incidence of air trapping, hypercarbia, and the risk of extrapulmonary leak such as pneumothorax, pneumomediastinum, and air dissection into the abdomen. Hypotension is a relative contraindication, and neonates with hypotension should be adequately volume-resuscitated and stabilized on vasopressors before the initiation of HFOV, as the transition to higher mean airway pressures may compromise right arterial preload [4].
Initial Settings
To improve alveolar recruitment upon the transition to HFOV, the initial mean airway pressure on HFOV is usually set 5 cmH2O higher than the last mean airway pressure during conventional ventilation. Lung hyperinflation should be avoided by cautious increase of mean airway pressure. The mean airway pressure is increased by increments of 1 cmH2O until arterial oxygenation improves. A chest radiograph is taken after transitioning to HFOV to confirm adequate lung expansion. Hyperinflation is manifested by lung expansion of more than nine ribs and flattened diaphragms. The FiO2 is usually set at 0.6–1.0 upon the transition to HFOV, and gradually weaned over time.
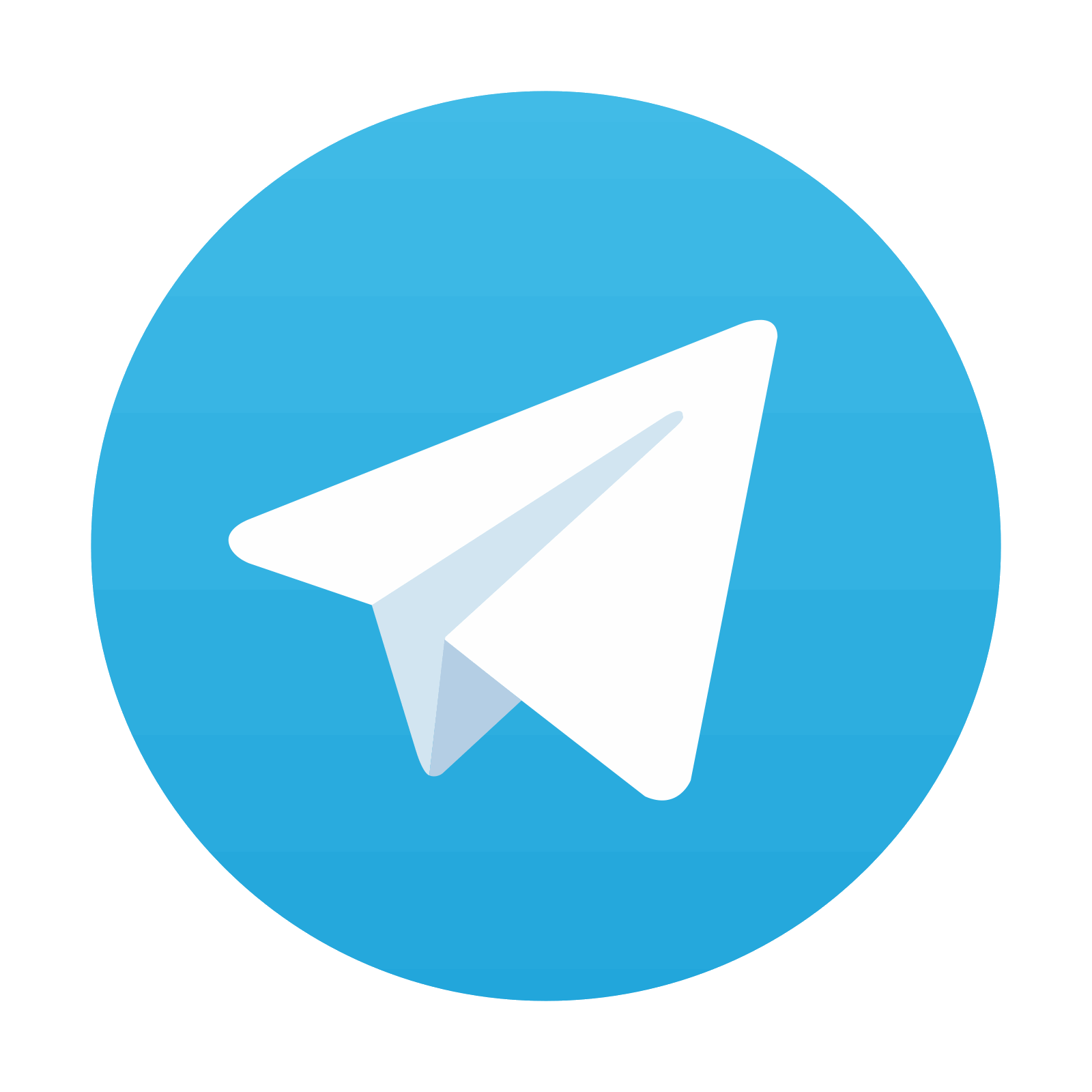
Stay updated, free articles. Join our Telegram channel
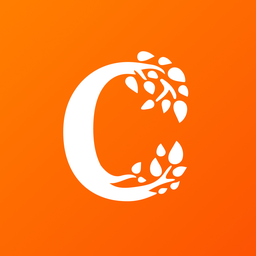
Full access? Get Clinical Tree
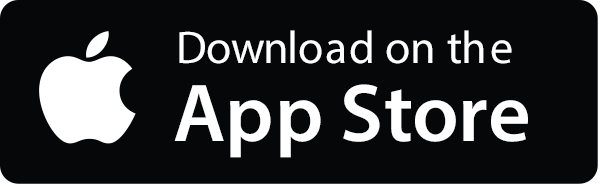
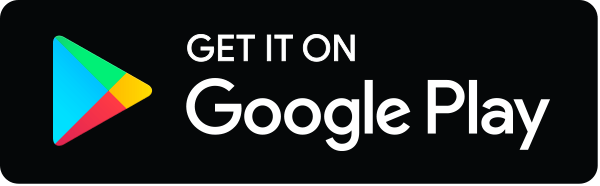
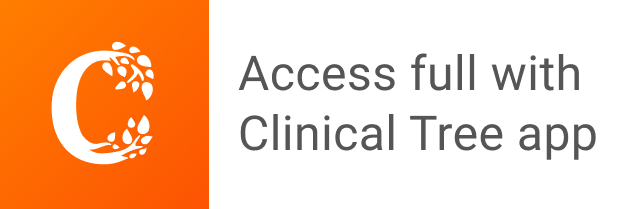