Management of fluids, blood products, and electrolytes in infants and children requires special attention to detail and constant vigilance. While the kidneys and neuroendocrine systems are responsible for fluid and electrolyte homeostasis, they are not fully developed at birth. Fluid compartment volumes throughout the body vary with age as well. As such, management of fluid resuscitation changes throughout infancy and childhood. In term infants, 17 percent will urinate in the delivery room and 90 percent will urinate in the first 24 hours.
Body Fluid Compartments
The body water compartment models that are most commonly utilized today are products of deuterium oxide dilution studies done over 60 years ago, which remain the best information available today. Total body water (TBW) rapidly changes throughout the first nine months of life. Term neonates have a TBW that is about 80 percent of total body weight while a very low birth weight (VLBW) preterm infant may have a TBW that is 90 percent of the total body weight [1].
At birth, extracellular volume (ECV) and intracellular volume (ICV) do not have the same proportional relationship that is observed after about three months of age into adulthood. In the peripartum period, ECV is best estimated as two-thirds of TBW, while ICV is one-third (an inverted relationship compared to what occurs after three months of age). In order for this relative fluid balance to change, there must be loss of fluid from the extracellular compartment, since the absolute ICV remains relatively steady. This loss of fluid is most pronounced during the first week of life: 5–10 percent of the birth weight of a healthy, term infant is lost. The fluid is lost in the form of transcutaneous losses and diuresis through natriuresis. Thus, fluid management must be more customized for the neonatal period while broader generalizations can be made later in infancy.
One important determinant of TBW, especially in the neonate, is the body fat percentage, since fat is largely anhydrous. While a VLBW preterm infant at 28 weeks gestation will have about 1 percent body fat, a term infant has approximately 10 percent body fat [2]. The result is that percentage body water is higher in premature infants, especially those that are VLBW. While this has widespread implications for pharmacology, electrolyte balance, and nutritional requirements, those topics are covered elsewhere. For the purposes of fluid management, it implies that fluid requirements may be greater than anticipated due to increased insensible losses, faster metabolic rate, immature kidney concentrating ability, and growth.
Formulas for accurate calculation of TBW are presented in Table 15.1; use age, height, gender, and weight for calculation of TBW.
Table 15.1 Total body water calculation based on gender, height, and weight
0 to 3 months of age: TBW = 0.887 × (Wt)0.83 |
3 months to 13 years: TBW = 0.0846 × 0.95 [if female] × (Ht × Wt)0.65 |
Children > 13 years: TBW = 0.0758 × 0.84 [if female] × (Ht × Wt)0.69 |
Intravascular Blood Volume
Plasma accounts for about one-fifth of ECV. Red blood cell volume is part of ICV. Rather than calculating intravascular volume each time from the TBW, there are accepted estimations of intravascular volume on an ml kg–1 basis that change based on age. Full-term neonates are estimated to have an intravascular blood volume of 90 ml kg–1 [4]. Preterm, VLBW, or critically ill infants have a higher percentage total body water and a higher intravascular blood volume, estimated to be about 100 ml kg–1 [5]. The estimated total intravascular volume continues to decrease on an ml kg–1 basis until adulthood, when 70 ml kg–1 is the generally accepted estimation of intravascular volume. Estimates of intravascular blood volume by age are presented in Table 15.2.
Table 15.2 Intravascular volume estimates based on weight
Age group | Intravascular volume |
---|---|
Preterm infants | 100 ml kg–1 |
Full-term neonates | 90 ml kg–1 |
Infants and toddlers | 80 ml kg–1 |
School-age children | 75 ml kg–1 |
Adolescents and adults | 70 ml kg–1 |
Electrolyte Balance
Sodium, as the primary determinant of serum osmolality, largely determines the distribution of water throughout the extracellular compartment, including intravascular volume. Homeostatic mechanisms to increase intravascular volume largely affect retention of sodium and water. Therefore, decreases in intravascular volume are brought about through loss of sodium and water.
In utero, the fetus has a high urine output, despite low blood flow to the kidneys and low glomerular filtration rate (GFR), due to almost no retention capability for sodium and water. By term, however, the kidneys are capable of conserving a great deal of the sodium and glomurulotubular balance is reached. All nephrons are developed fully by 36 weeks’ gestation, but renal blood flow (RBF) is still not high enough to produce a GFR that is consistent with adult GFR.
In preterm infants, however, the nephrons have not yet fully matured and glomerulotubular balance is not yet intact. Renal blood flow does increase postpartum and GFR increases, but the nephrons are not capable of balanced resorption of sodium. Therefore, sodium is not reabsorbed in proportion with GFR. There is also a poor response of the distal tubule to mineralocorticoids in preterm infants [7]. The result is natriuresis and diuresis, increasing their susceptibility to hyponatremia and hypovolemia. Other mechanisms of kidney immaturity result in the neonate being incapable of adult levels of urine concentration until approximately two years of age [8].
The neonatal kidney’s diluting capacity matures more rapidly. During the first 24 hours of life a neonate may be unable to increase water excretion appropriately [9]. Diluting capacity is fully mature about one month postpartum.
Fluid Requirements for Maintenance and Resuscitation
In order to calculate the fluid needed for any given patient, the anesthesiologist must keep in mind several issues. Fluid deficit, metabolic requirements, insensible loss, blood loss, and other outputs must all be considered in order to make an estimation of the fluid requirements of a particular patient. No formulas can precisely predict the fluid required in any given patient undergoing any particular procedure. Rather, fluid management often becomes part of the art of anesthesia.
The long used formula for estimating the maintenance fluid requirements for a child is derived from a study by Holliday and Segar in 1957 [10]. This formula provides the starting point for the calculation of fluid deficit and basic fluid requirements in healthy children. It was never intended to be the sole guidance for fluid administration to every hospitalized child, but many have expanded the conclusions in this paper beyond their original intent. This resulted in another paper by the same authors in 2004 that suggested that resuscitation fluid be isotonic and given in boluses as initial resuscitation while maintenance fluid should be given that is hypotonic to prevent excess salt wasting [11].
When maintenance fluid estimates were initially calculated, they were based on metabolic rate calculations and obligatory urine output estimates. However, the critically ill patient can have severe perturbations in both metabolic rate and urine output. The maintenance fluid estimates used today are still derived from Holliday and Segar. The 4–2–1 rule of maintenance fluid calculation is that there should be 4 ml kg–1 h–1 for the first 10 kg of body weight, an additional 2 ml kg–1 for the next 10 kg of body weight, and an additional 1 ml kg–1 for the remainder of weight over 20 kg. The suggested fluid would be a hypotonic, glucose-containing solution after initial resuscitation has been undertaken, since daily requirements for sodium, chloride, and potassium were also calculated based on metabolic rate and obligatory urine loss.
It is generally accepted in the operating room that metabolic rate falls under anesthesia, especially if hypothermia is observed or induced. Therefore, it is a common practice to decrease the rate of glucose administration in the operative environment, compared with glucose administration to a hospitalized child in another setting. Furthermore, the stress response to surgery further increases serum glucose levels. This topic is covered in greater detail in Chapter 6, Glucose Control.
While the 4–2–1 rule is generally used to calculate the fluid deficit of a child, isotonic fluid solutions should be used for resuscitative efforts. The most common fluid deficit encountered in the operating room is the fasting time for those children presenting to surgery from home. It is calculated by multiplying the maintenance fluid rate and total fasting time for liquids [12]. This deficit should be replaced over the first portion of a long case, but can be replaced faster in patients in whom there are no reasons to suspect heart disease. More specifically, it has been suggested that the first half of the deficit be replaced over the first hour of surgery and the other half over the following two hours. A simplification of this is to assume an average fasting time of 6–8 hours and administer to children under four years of age 25 ml kg–1 and children four years or older 15 ml kg–1 of isotonic solution [13]. However, current common practice is to give maintenance IV fluids to inpatients awaiting surgery and to encourage outpatients presenting for surgery to drink clear liquids until two hours before surgery, based on the ASA guidelines for preoperative fasting [14]. Therefore, those bolus estimations are probably an overestimation of fluid deficit.
Since the 4–2–1 rule was calculated based on estimations of metabolism and did not account for the body’s compensatory mechanisms for fasting, it may be that this is also an overestimation of the actual fluid deficit. In healthy adults, there is evidence that their intravascular volume is maintained even with prolonged fasting times, but this is probably not the case with neonates [15].
In some conditions, increased fluid loss is obvious, such as in cases of diarrhea or vomiting. But, some fluid deficits are more insidious, such as encountered with burns or with significant capillary leak that can occur with strangulated hernia, gastroschisis, or sepsis. Since the fluid that needs to be replaced in these scenarios is most similar to the extracellular environment, the resuscitation is best accomplished with crystalloid solutions most similar in osmolality and solute makeup to ECV, such as lactated Ringer’s. Some have recommended initial resuscitation volumes of approximately 40 ml kg–1 for cases of severe dehydration [16]. In cases where renal impairment is suspected, potassium-free fluids may be indicated.
The low birth weight (LBW) and VLBW infants will have three well-described phases of urine output. First, the first day of life is a low intake and low urine output state – therefore body weight is stable. Days two and three of life are characterized by diuresis, independent of any fluid intake. Then days four and five are when urine output begins to vary more directly with fluid intake. Premature infants who are treated with excess fluids have a higher incidence of respiratory distress, necrotizing enterocolitis, left ventricular failure, and patent ductus arteriosus [17]. Because renal function, insensible water losses, and metabolism change rapidly in the newborn, it can be difficult to apply a generic formula to all neonates for daily fluid requirements. Based on practical experience, neonatologists have developed recommendations for fluid management in the first seven days of life (Table 15.3).
Table 15.3 Daily fluid requirements during first week of life (ml kg–1 day–1)
Birth weight | Day 1 | Day 2 | Day 3 | Day 4 | Day 5 | Day 6 | Day 7 |
---|---|---|---|---|---|---|---|
<1000 g | 80 | 100 | 120 | 130 | 140 | 150 | 160 |
1000–1500 g | 80 | 95 | 110 | 120 | 130 | 140 | 150 |
>1500 g | 60 | 75 | 90 | 105 | 120 | 135 | 150 |
Even though diuresis and natriuresis are concerns during the neonatal period, it is relatively easy to encounter fluid overload in the operative setting. From a practical standpoint, use of large-bore IV tubing may result in flush volumes that are larger than the hourly maintenance fluid requirements for a neonate. Use of heated humidifiers in the ventilator circuit may deliver a large, insensible volume of fluid that is readily absorbed through the lungs. Intravenous solutions hung to gravity, even with a volumetric burette, are easily administered in full before their rates of infusion are realized by the provider.
From a practical standpoint, neonates undergoing surgical procedures are likely to be inpatients. They have IV access and are likely to be receiving a hypotonic, glucose-containing solution for maintenance. Others will arrive to the operating room on total parenteral nutrition (TPN) with or without lipid infusions. Lipid infusions can almost always be safely discontinued for surgery and should be discontinued in most cases (burns may represent an important exception to this rule). A general approach to the glucose management and to the maintenance fluid management is to maintain the infusion either at maintenance rates or half maintenance rates by the 4–2–1 rule. All other fluid given for insensible losses, volume resuscitation, and blood loss should be isotonic or hypertonic crystalloid solutions or colloid solutions like albumin, in order to avoid hyperglycemia [18]. Maintenance of this hypotonic solution also ensures that there is at least some free water available for elimination of other solute load from resuscitation, since neonates and infants are unable to concentrate urine at adult levels.
Frequent intraoperative glucose monitoring is indicated. Glucose management may also be complicated by the large amounts of dextrose in stored blood products. TPN and maintenance fluids may also contain potassium, which may become a concern if rapid transfusion of blood products is anticipated.
Fluid resuscitation may be needed due to intraoperative losses. There is great ongoing loss when the exposed surface area, as a percentage of the child’s total surface area, is high. The classic example is dressing changes for burns. While the burn is covered in wet gauze, there is little ongoing loss to the environment. When the burn is exposed to the operating environment, the losses can be extraordinary. While this direct evaporative loss may not be as important in older children, this can represent an enormous loss of fluid from an infant.
There can be great variations in capillary leak induced by surgical injury, and this amount of fluid can also be very difficult to quantify. This fluid is generally described as third space losses, which is a shift of fluid into a nonfunctional extracellular space that is not in equilibrium with the intravascular compartment [19]. It was suggested that the greater the trauma related to the surgery, the greater this third space shift would become. Furthermore, redistribution of blood flow, induced by anesthetics, patient positioning, venous stasis, and temperature regulation may lead to greater relative intravascular volume depletion even in the most minimally invasive cases.
Ongoing blood loss is the most direct cause of intravascular volume depletion. As a general rule, 3 ml of isotonic crystalloid solution should be provided for each 1 ml of blood loss. However, between dilution of existing intravascular blood and even moderate amounts of blood loss, neonates have a limited capacity for replacement of blood volume with crystalloid alone. Colloid may also be used, 1 ml for each 1 ml of blood loss [20]. However, the most direct replacement of blood loss is with blood products, to be discussed later in this chapter.
There have been some studies to suggest fluid management for a variety of cases. One such case is gastroschisis, in which fluid requirements are quite high in the first day of life. Where the neonate usually requires minimal fluid during the first 24 hours of life, neonates with gastroschisis require 150 ml + 35 ml kg–1 over the first 24 hours, 75 percent of which was crystalloid and 25 percent of which was colloid in this particular study [21]. Depending on the level of trauma expected in a particular surgical procedure, 1 ml kg–1 h–1 to 15 ml kg–1 h–1 has been suggested for replacement of continued intraoperative losses. For premature neonates undergoing laparotomy for necrotizing enterocolitis, up to 50 ml kg–1 h–1 has been utilized in the literature [22]. In a more general sense, small surgical procedures with mild tissue trauma may have third space and evaporative losses of about 3–4 ml kg–1 h–1, while large abdominal procedures may lead to more significant losses of 10 ml kg–1 h–1 or more [23].
Colloid and Hypertonic Solutions
Colloids are a valuable adjunct for intravascular volume replacement. As they generally remain intravascular for longer, the amount of colloid needed for the same intravascular volume replacement versus isotonic crystalloid is less. Colloids that are generally available are albumin, as 5 percent or 25 percent solution, and hydroxyethyl starches (HESs). While dextrans are commercially available, they are not often used because of their negative coagulation effects and increased risk of anaphylaxis [24]. Gelatins are commercially available as well, but their use was discontinued in the United States in 1978 because of hypersensitivity reactions [25].
Albumin 5 percent solution is osmotically equivalent to an equal volume of plasma. Thus, the 25 percent solution is five times more osmotically active. Otherwise stated, 100 ml of 5 percent albumin increases plasma volume by 100 ml, while 25 percent albumin may increase plasma volume by 300–500 ml [26]. The volume-limiting benefit of albumin may not be as certain in the setting of significant tissue trauma, as capillary leak may allow albumin to translocate extravascularly and osmotically draw fluid into the interstitium.
Albumin use in the pediatric population is perhaps most well-studied in infants. Albumin has long been considered the standard colloid against which all others are measured and, as of 2001, was still the most commonly used colloid in children [27,28]. Its long history in clinical use is likely due to its benign side-effect profile. It has been shown in adults to have minimal effects on the coagulation cascade as long as total dose is below 25 percent of blood volume [29] and carries no risk of disease transmission [30]. It has almost no contraindications aside from increased mortality rates when used in adults for initial resuscitation after traumatic brain injury.
The use of albumin intraoperatively versus crystalloid has really only been studied in children undergoing cardiopulmonary bypass [31,32]. Hence, there is not much guidance for its use other than generic dosing, which is 10–20 ml kg–1 for 5 percent albumin [33] and 2.5–5 ml kg–1 for 25 percent albumin [34]. In hypoalbuminemic patients who are also hypotensive, replacement of albumin may be calculated by the following equation [35]:
HESs are synthetic colloids that may be used for volume expansion. They are osmotically active and too large for diffusion through most capillary beds into the intravascular space. They are degraded in the bloodstream by amylase and ultimately eliminated renally. Depending on the HES solution, they have a half-life of 2–6 hours [36]. The side-effects include impairment of the coagulation cascade, renal toxicity, and pruritus [37].
There are three HES solutions currently available in the United States: Hespan, Hextend, and Voluven. Each are 6 percent HES solutions, but they have different molecular structures that change the half-life of their effect on volume as well as their tendency to affect coagulation parameters. Hextend and Hespan have a longer half-life of 5–6 hours, and Hextend has fewer effects on the coagulation cascade than Hespan [38]. Voluven has minimal effects on the coagulation cascade, but boasts a half-life of only 2–3 hours [39]. Voluven has no renal side-effects [40].
There are several studies of HES solutions in pediatric patients, demonstrating their safety and capacity for volume expansion. Importantly, a study in 26 neonates undergoing central line placement revealed there was no increase in creatinine or bleeding with the use of HES versus albumin [41]. Another study of 316 children with normal baseline coagulation and renal function were infused 5–15 ml kg–1 of Voluven and no serious adverse reactions such as anaphylaxis, renal failure, or clotting disorder were observed [42]. These, among other studies, lend credit toward HES use for volume expansion in even the smallest of patients. The product inserts for Hextend and Hespan suggest that the approximate maximum dose that should be used is 20 ml kg–1 for adults, but that pediatric trials are unavailable [43]. The product insert for Voluven indicates a maximum dose of 50 ml kg–1 regardless of age group [44]. Large-scale adult studies have questioned renal toxicity and mortality rates in patients treated with HES. As a result of these studies, the US Food and Drug Administration (FDA) and several international organizations have recommended limiting the use of HES [45].
Hypertonic saline is increasing in use in the perioperative setting across many practices. It is a valuable tool in resuscitation because of its hypertonicity and, therefore, its ability to increase plasma volume. Hypertonic saline has more traditionally been used for the treatment of acute or severe hyponatremia and cerebral edema in neurotrauma. There have been two studies in children that revealed increased cerebral perfusion pressure in the three days after traumatic brain injury when using hypertonic saline versus lactated Ringer’s solution [46,47]. The use of hypertonic saline in children was not associated with renal failure in a previous study of its effects on intracranial pressure [48]. Many institutions have a limit on what rate of infusion may be used for various concentrations of hypertonic saline, but this is poorly studied and generally sodium levels should be followed during therapy and should not exceed 155 mEq/L.
There are no reports in the literature of normonatremic patients suffering from central pontine myelinolysis as a result of hypertonic saline use. If 3 percent NaCl is used, the chloride load can be quite high and this leads to a non-anion gap metabolic acidosis. However, many institutions make buffered solutions in which acetate is used to buffer some of the sodium in solution rather than all chloride. Acetate is then metabolized to bicarbonate, which helps offset the metabolic acidosis often seen in children receiving large chloride loads. In adult trauma literature, there is evidence that hypertonic saline modulates the immune system dysfunction that is related to trauma [49].
Hypertonic saline may induce vascular damage when it is injected into small peripheral veins. Up to 3 percent hypertonic saline has been used regularly without a central line. When mixed with red blood cells for transfusion, hypertonic saline can induce hemolysis. Similarly, the hypertonicity of fluid may cause tissue damage if a significant infiltration occurs. There are only a few case reports of successful use of hypertonic saline in neonates, and it should only be considered in cases of extreme hyponatremia.
Postoperative Fluid Management
Postoperative administration of hypotonic fluid is common [50]. The traditional teaching that led to this practice is based on the aforementioned work by Holliday and Segar. Neonates may not be capable of eliminating any excess solute provided due to immature urine concentrating ability. Thus, the traditional practice has been to administer hypotonic fluid to children postoperatively.
Syndrome of inappropriate antidiuretic hormone secretion (SIADH) is a common response to the stress state induced by surgery. Taken with the fact that “routine” postoperative labs are not typically obtained and hypotonic fluids are routinely administered, hyponatremia may be a real risk to patients postoperatively. In fact, there are reports of significant harm coming to children in that situation [51]. Hyponatremia is difficult to identify clinically due to the general symptoms encountered: nausea, vomiting, headache, and lethargy. However, a meta-analysis comparing hypotonic fluids versus isotonic fluids in all hospitalized children showed the odds of developing hyponatremia after administration of hypotonic IV fluid were 17 times greater than isotonic fluid, and resulted in greater patient morbidity [52]. Another recent meta-analysis confirmed that postoperative children receiving hypotonic fluids were much more likely to develop hyponatremia than those receiving isotonic fluid [53].
In a clinical trial looking at the type of maintenance IV fluid to administer postoperatively to pediatric patients, excluding children under six months of age, it was concluded that the administration of isotonic fluid, rather than traditional hypotonic fluids, decreases the risk of postoperative hyponatremia. There was no difference in postoperative ADH levels or in risk of adverse events between the groups that received isotonic or hypotonic fluids postoperatively. Isotonic fluids did not increase the risk of hypernatremia [54]. While there is not currently enough evidence to completely change postoperative fluid administration practices, there may be additional evidence in the future that hypotonic fluids are not desirable postoperatively. Because of the unpredictable response of neonatal patients to hypotonic and isotonic solutions, it is recommended that serum electrolytes be measured in post-surgical neonates receiving intravenous hydration.
Physiologic Anemia and Anemia of Prematurity
At birth, the normal neonate has a hemoglobin concentration of approximately 17 g dl–1, a hematocrit of 55 percent, and large red blood cells. Within one week, the hemoglobin and hematocrit begin to fall from approximately 17 g dl–1 at birth to 9–11 g dl–1 at 8–12 weeks. This expected decrease in hemoglobin and hematocrit is termed physiologic anemia of infancy. Although the drop seems dramatic, this anemia is asymptomatic in the healthy neonate.
Decreased erythropoiesis early in the neonatal period is largely responsible for this physiologic anemia. Erythropoietin is made in the fetal liver and later in the kidneys. It is synthesized in response to low oxygen tension. When oxygen tension rises at birth, erythropoietin synthesis decreases. In the first 4–6 months, hemoglobin F is also replaced with hemoglobin A and levels of 2,3-diphosphoglycerate (2,3-DPG) climb. The change in conformation of the predominant hemoglobin along with more 2,3-DPG increases the P50 from 19 mmHg to 27 mmHg and shifts the oxyhemoglobin dissociation curve to the right [55]. As this shift occurs, the increased oxygen delivery to tissues continues to contribute to decreased erythropoiesis. As red blood cells lose the enzymatic function needed to maintain their deformable membrane, they become fragile and destructible. In the setting of decreased erythropoiesis, destroyed red blood cells are not replaced, and anemia further ensues.
At 8–12 weeks, oxygen needs begin to exceed oxygen delivery. In response to lower oxygen tension, erythropoietin gene expression increases, erythropoiesis resumes, and hemoglobin and hematocrit levels rise to near adult levels over the next six months.
In preterm infants, hemoglobin decreases weeks earlier, falls further, and takes longer to recover without treatment. During anemia of prematurity, hemoglobin reaches a nadir of 7–8 g dl–1 by 3–6 weeks of age. Unlike the physiologic anemia of infancy, this is often symptomatic, causing arrhythmias, respiratory distress and apnea, and inadequate weight gain. Erythropoietin synthesis has not yet converted from the liver, where EPO gene expression is less responsive to hypoxia, to the kidney, where it is more responsive. As a result, in spite of symptomatic low oxygen tension, erythropoiesis remains decreased and anemia continues.
While healthy term infants require no treatment for physiologic anemia, preterm neonates often receive transfusions for anemia of prematurity. Studies of liberal and restrictive transfusion parameters in preterm neonates have been conflicting. While some have shown no increased morbidity or mortality with restrictive transfusion therapy [56], at least one has shown more frequent adverse neurologic outcomes with a restrictive strategy [57]. In the absence of an established optimal hemoglobin target, premature neonates should be transfused based on their clinical condition while taking into consideration baseline hemoglobin, increased oxygen affinity of residual Hgb F, and expected blood volume for age.
Transfusion Guidelines
Very young children have smaller total blood volume, decreased production of erythropoietin in response to anemia, and immature humoral systems that render them less capable of forming antibodies to antigenic red blood cells [58,59]. Because of these physiologic differences, very young children are transfused under different guidelines than those greater than four months. Historically, in fact, premature neonates were transfused simply to replace blood loss from frequent phlebotomy. While this is no longer recommended, patients in this age group are still among the most frequently transfused in the hospital [60,61].
The following are current guidelines for transfusion of red blood cells in patients less than four months of age [62]:
Hct <20 percent with low reticulocyte count and symptoms of anemia.
Hct <30 percent with an infant on <35 percent hood O2, O2 per nasal cannula, CPAP, or IMV with mechanical ventilation with mean airway pressure <6 cmH20.
Hct <30 percent with an infant with significant apnea, bradycardia, tachycardia, tachypnea, or poor weight gain.
Hct <45 percent with an infant on ECMO or with congenital heart disease.
Hct <35 percent with an infant on >35 percent O2 or on CPAP/IMV with mandatory ventilation with mean airway pressure ≥ 6 cmH2O.
The indications for transfusion of red blood cells to older infants and children are similar to those in adults [62]:
Emergency surgery in patients with significant preoperative anemia.
Preoperative anemia when other corrective therapy is not available.
Intraoperative blood loss ≥15 percent total blood volume.
Hct <24 percent in perioperative period with signs and symptoms of anemia while on chemo/radiation or with chronic congenital or acquired symptomatic anemia.
Acute blood loss with hypovolemia not responsive to other therapy.
Hct <40 percent with severe pulmonary disease or ECMO.
Sickle cell disease with cerebrovascular accident, acute chest syndrome, splenic sequestration, recurrent priapism, or preoperatively to reach Hb 10 g dl–1.
In the intraoperative period, a calculation of maximum allowable blood loss is often useful in determining when to transfuse. This method uses estimated blood volume, initial hematocrit, and lowest allowable hematocrit to determine how much blood a patient can lose before transfusion is necessary [63]:
The decision to transfuse cannot be based on the predetermined lowest allowable hemoglobin alone, however. In the setting of rapid blood loss, expected ongoing blood loss, and in the face of clinical signs of decreased oxygen-carrying capacity, it may be necessary to transfuse at higher hemoglobin values.
Many studies have investigated transfusion triggers in critically ill adults, but far fewer have been performed in pediatric patients. In 1999, a landmark trial by Herbert et al. found a higher in-hospital mortality rate and higher estimates of severity of multiple organ dysfunction and nosocomial infections in adult patients transfused for a hemoglobin less than 10 g dl–1 as compared to those transfused for a hemoglobin less than 7 g dl–1 [64]. There was no difference in 30-day mortality between the groups.
A study performed in 2007 of critically ill pediatric patients found similar results. Those transfused according to a liberal (transfusion for hemoglobin less than 9.5 g dl–1) strategy received 44 percent more transfusions than those transfused according to a restrictive strategy (transfusion for hemoglobin less than 7 g dl–1); but there was no significant difference in percentage of patients with new or worsening multiple organ dysfunction syndrome or death between the two groups [65]. This suggests that a transfusion threshold hemoglobin of 7 g dl–1 may be appropriate in critically ill children who are not actively bleeding.
In any case, the indication for red blood cell transfusion is to increase oxygen-carrying capacity in order to ensure adequate oxygen delivery to tissues. We see this in the aforementioned transfusion criteria, which highlight clinical scenarios in which tissue oxygen delivery is compromised or at risk. Therefore, the assessment of a child’s clinical picture is as important as any laboratory value in making the decision to transfuse.
Unique Populations
Congenital Heart Disease
Pediatric patients with uncorrected cyanotic congenital heart disease require more hemoglobin – higher oxygen-carrying capacity – to ensure adequate oxygen delivery to tissues. Intracardiac shunts result in the delivery of desaturated blood to tissues and polycythemia develops due to increased erythropoietin in response to low tissue oxygen tension. Although it is common practice to maintain hemoglobin levels between 13 and 18 g dl–1 in these patients, there are few data to support this [66]. As in all cases, an assessment of the child’s clinical picture must be used to determine when to transfuse.
Sickle Cell Disease
Sickle cell disease is a hereditary hemoglobinopathy that results from a single point mutation on the hemoglobin β-A gene, the gene that codes for beta globulin chains of hemoglobin A. This mutation results in production of hemoglobin S, which deforms or sickles the erythrocyte when deoxygenated [67]. Clinically, this sickling results in chronic hemolytic anemia, intermittent vaso-occlusion causing tissue anoxia and severe pain, and progressive organ damage. When the infant is around 4–5 months of age, the baby or fetal hemoglobin is replaced by sickle hemoglobin and the cells begin to sickle. Often the first manifestations of sickle cell disease in the infant is painful swelling of the hands and feet.
In 1955, the first major review of the anesthetic implications of sickle cell disease found a high rate of serious and even potentially fatal exacerbations of the disease after surgery [68].
Patients with sickle cell disease continue to have a 10 percent perioperative mortality rate and a 50 percent rate of serious disease-related postoperative complications – acute chest syndrome, painful crisis, and neurologic events [65]. Diluting hemoglobin S concentrations to less than 30 percent has been advised by some as a way to decrease the perioperative morbidity and mortality [69]. However, a study comparing a restrictive transfusion strategy (transfusion for hemoglobin less than 10 g dl–1) with a liberal strategy (transfusion to dilute hemoglobin S to less than 30 percent) found no difference in the incidence of aforementioned major perioperative complications between the two groups in spite of the fact that the restrictive transfusion group had hemoglobin S concentrations of 59 percent compared with the liberal group’s 31 percent [65]. These results suggest that diluting the hemoglobin S concentration may not reduce the rate of complications perioperatively.
The next question concerns the lowest acceptable preoperative hemoglobin in the sickle cell patient. A recent randomized trial compared preoperative transfusion with no preoperative transfusion in children and adults with hemoglobin SS or S-β-thalassemia sickle cell disease undergoing low and medium risk surgery [65]. The trial was terminated when the investigators found a marked increase in severe adverse events – primarily acute chest syndrome – in the group who was not transfused preoperatively. They concluded that patients with a preoperative hemoglobin less than 9 g dl–1 should be transfused to decrease the risk of acute chest syndrome in the perioperative period.
Certain types of operations and patient characteristics seem to be associated with an increased incidence of perioperative complications in patients with sickle cell disease. A review of 1079 procedures found no complications with tonsillectomy and adenoidectomies, but complication rates of 16.9 percent for hysterectomies and Cesarean sections and 18.6 percent for dilation and curettage [65]. Increased age, recent complications, pregnancy, infection, and inpatient status also seem to be associated with increased sickle cell-related morbidity in the perioperative period [70]. However, in children undergoing cholecystectomies, a younger age increased the risk of developing acute chest syndrome. [71]
Therefore, type of surgery and patient characteristics must be taken into account along with preoperative hemoglobin in the decision to transfuse the sickle cell patient. Finally, the benefits of red blood cell therapy in sickle cell disease patients much be weighed with the costs of repeated transfusion, including iron overload and the risk of alloimmunization.
Thalassemia
The beta thalassemias are a group of inherited disorders characterized by decreased production of alpha globulin chains of hemoglobin [72]. Severe anemia occurs due to hemolysis and ineffective erythropoiesis, and patients with severe disease are transfusion-dependent [73]. Maintenance of hemoglobin levels of 9–10 g dl–1 allows for normal growth and development and reduces bone deformities and hepatosplenomegaly from extramedullary hematopoiesis [65,74]. Unfortunately, repeated transfusions lead to iron overload. In fact, cardiac death from iron overload is the primary cause of death in this hemoglobinopathy; patients must be treated with chelation therapy beginning in childhood [75].
Preparation of Products
Cytomegalovirus-Seronegative Blood Components
Cytomegalovirus rarely causes serious illness in the immunocompetent host [76], but immunosuppression can allow for uncontrolled viral replication and serious disease [77]. Infants born to seronegative mothers have historically been transfused with CMV-seronegative blood. These recommendations were based on a study of preterm neonates that showed that transfusing CMV-seronegative blood products to multi-transfused low weight (<1200 g) neonates of seronegative mothers reduced the risk for CMV infection [78].
Low birth weight neonates, HIV-infected patients, recipients of seronegative allogeneic organ or hematopoietic stem cell transplants, pregnant women, and those receiving intrauterine transfusions should receive CMV-negative products. Patients with Hodgkin and non-Hodgkin lymphoma, recipients of immunosuppressive therapy, candidates for autologous hematopoietic stem cell transplantation, and those with hereditary or acquired cellular immunodeficiency can be considered for CMV-negative red blood cells.
Leukocyte Reduction
Leukocyte reduction is the filtration of leukocytes from donor blood products prior to transfusion. Over 80 percent of the blood products transfused to pediatric patients in the United States have been leuko-reduced in spite of few studies demonstrating benefit [79]. The process reduces the transmission of CMV [65], HLA alloimmunization, and febrile hemolytic transfusion reactions. Patients with congenital hemolytic anemias (sickle cell disease and thalassemia) and hypoproliferative anemias who are likely to need multiple transfusions should be transfused with leuokocyte-reduced red blood cells in order to prevent alloimmunization [65]. Because of their immature immune systems, however, neonates less than four months of age rarely become alloimmunized. They also infrequently suffer from febrile transfusion reactions. As such, leukocyte reduction of blood products for neonates remains controversial. A study of LBW (<1250 g) premature infants before and after implementation of universal leukocyte reduction found no difference in mortality or rate of bacteremia. There was, however, a decrease in retinopathy of prematurity, bronchopulmonary dysplasia, and length of hospital stay after leuko-reduction [65]. This evidence supports continued leukocyte reduction for the youngest, smallest patients.
Irradiation
While leukocyte reduction may also decrease the risk of transfusion-associated graft-versus-host disease (TA-GvHD), blood products must be irradiated in order to inactivate T-cells in the donated blood product and prevent the development of TA-GvHD. TA-GvHD occurs when transfused T-lymphocytes attack host HLA-incompatible lymphoid tissue and host T-lymphocytes fail to mount an appropriate response [80]. This can occur not only in immunocompromised patients but also in immunocompetent recipients who share HLA antigens with the donor [65]. The disease carries a high rate of fatality from infection as the host’s bone marrow become hypoplastic [81].
Although it is not an absolute indication, neonates less than four months of age who may have undiagnosed cellular immune deficiency often receive irradiated fresh blood products. Low birth weight neonates (<1200 g), neonates receiving exchange transfusion, and those on extracorporeal membrane oxygenation should receive irradiated products. Patients with congenital cell-mediated immunodeficiencies, Hodgkin disease, acute lymphocytic leukemia, those receiving purine analogue therapy, and patients receiving direct donations from relatives or HLA-matched donors must have irradiated products, as should patients receiving hematopoietic stem cell transplants, intrauterine or postintrauterine transfusions, and immunocompromised organ transplant recipients [65].
Washing
Washing blood products removes or reduces plasma, anticoagulant-preservative solutions, and high levels of potassium. While it is not used routinely, children and infants who may be predisposed to hyperkalemia may preferentially receive washed products. In addition, the process of irradiation increases the potassium in the blood product, which should then be washed before transfusion to infants to avoid hyperkalemia.
Age of Blood
While some institutions transfuse red blood cell products less than seven days old to neonates, others use a dedicated unit until the expiration date to reduce donor exposure. A study comparing the use of fresh versus standard-issue red blood cell transfusion in premature infants found no difference in outcome [65].
Platelets
Platelets are necessary to maintain an intact endothelial barrier to prevent spontaneous bleeding and, in higher levels, for hemostasis after vascular injury to control surgical bleeding. Patients of all ages are at risk for spontaneous hemorrhage at platelet counts <10 000/μl [65]. If active bleeding is occurring or an invasive procedure is imminent, platelet counts of 50 000/μl are usually required [82]. For those patients with platelets counts between 50 000/μl and 100 000/μl, platelets do not need to be given prophylactically but should be immediately available in case of microvascular bleeding during invasive procedures [66]. In some clinical settings, in which even small amounts of bleeding could be catastrophic, the platelet transfusion trigger is higher [83]. In neurosurgery, for example, patients may be transfused for platelet count <100 000/μl, even without active bleeding [63].
Critically ill preterm infants are a population that deserves particular attention here. These patients have an immature subependymal matrix prone to rupture, putting them at risk of intracranial hemorrhage [61,84]. Specific transfusion guidelines for these infants are locally defined, but recommendations suggest transfusing them when platelet counts fall below 60 000/μl [85]. However, a randomized controlled trial comparing transfusion to platelet count >150 000/μl versus >50 000/μl found no significant difference in the incidence of intracranial hemorrhage between the groups [65]. Therefore, as with all transfusions, the risks and potential benefits must be weighed on a case-by-case basis after careful clinical assessment.
Platelets can be collected in one of two ways. They can be derived from whole-blood donations and then pooled with 5–7 other donors. Alternatively, they can be collected from a single donor through apheresis. The later method produces approximately the same number of platelets but reduces exposure to multiple donors [86]. Plasma-incompatible, whole-blood-derived platelets contain very small volumes of plasma compared to the total plasma volume of an adult patient. As such, these transfusions do not lead to clinically significant hemolysis. However, apheresed platelets and whole-blood platelets given to small patients should be ABO compatible to avoid a hemolytic reaction. Rh antigens are not expressed on platelets, so Rh(D) matching is not necessary for apheresis platelets. Whole-blood-derived platelets contain enough red blood cells to cause Rh alloimmunization, so platelets from Rh(D) negative donors are preferentially given to Rh(D) negative recipients of childbearing age.
There are several important logistical considerations in the transfusion of platelets. Pediatric patients are typically administered 10–15 ml kg–1 to increase their platelet count by 30 000 to 90 000/μl. However, this dose is currently being studied and discussed. In light of recent evidence suggesting lower doses may achieve adequate hemostasis, the dose may be reduced in the future [87,88]. When given in anticipation of an invasive procedure, platelets should be transfused just prior to the procedure. They should only be filtered by large-pore filters (>150 μm) and should be stored at room temperature. Recent evidence suggests, though, they may be safely infused through a warmer during transfusion [89].
Fresh Frozen Plasma and Cryoprecipitate
Fresh frozen plasma (FFP) is the fluid portion of whole-blood containing all of the coagulation factors in normal concentrations. Fresh frozen plasma is indicated to replace factors during massive blood transfusion, to correct a severely prolonged prothrombin time prior to an invasive procedure or during active bleeding, to emergently reverse warfarin, or as replacement therapy when specific factor concentrates are not available. Preterm infants and neonates most often receive FFP to treat factor deficiencies from vitamin K deficiency and in cases of hemorrhagic disease of the newborn in which coagulation factors are depleted. Unfortunately, it is also often transfused without evidence-based justification in cases where no coagulopathy exists and a colloid would be more appropriate for resuscitation [90].
Cryoprecipitate is prepared from FFP by thawing the product and separating the precipitated protein. This contains 20–50 percent of the Factor VIII from the original FFP, von Willebrand factor, approximately 250 mg of fibrinogen, and Factor XIII. It is indicated in cases of hypofibrinogenemia, dysfibrinogenemia, or Factor XIII deficiency, but is no longer used to treat hemophilia A or von Willebrand disease [91,92]. Infants usually only require 1 unit and children 1–2 units per 10 kg to raise fibrinogen 60–100 mg dl–1.
Coagulation Disorders and Their Specific Treatment
About 1 in 5000 males is born with hemophilia every year [93]. The severity of bleeding with these coagulopathies is proportional to the degree of factor deficiency [94]. Those with milder disease may only bleed with trauma or surgery, but children with more severe disease may bleed spontaneously [95]. Children with these inherited factor deficiencies were historically treated with pooled plasma, putting them at high risk for contracting viral hepatitis and HIV. Transmission rates have now been greatly reduced, however, due to more effective virus removal and inactivation and the use of recombinant factors. Patients with hemophilia A are treated with Factor VIII. Similarly, children with the much less common hemophilias B, or Factor IX deficiency, are transfused with recombinant Factor IX or purified Factor IX products.
Table 15.4 lists specific guidelines for factor repletion in hemophilia A and B.
Table 15.4 Specific guidelines for factor repletion in hemophilia A and B
Site of hemorrhage | Target Factor VIII or IX level (percent) | Treatment duration (days) |
---|---|---|
Muscle | 30–50 | 1–2 |
Joint | 50–80 | 1–2 |
Gastrointestinal tract | 40–60 | 10–14 |
Oral mucosa | 30–50 | 2–3 |
Epistaxis | 30–50 | 2–3 |
Hematuria | 30–100 | 1–2 |
Retroperitoneal | 80–100 | 7–10 |
Central nervous system | 80–100 | 14 |
Trauma or surgery | 80–100 | 14 |
Von Willebrand disease (vWD) results from an abnormal amount, structure, or function of von Willebrand Factor (vWF), a glycoprotein that acts to facilitate platelet adhesion and transport Factor VIII, preventing its degradation. It was once thought to be more common than hemophilia, but may be as rare as 1 case per 10 000 people. Children with vWF often suffer from bruising, epistaxis, and menorrhagia due to impaired platelet adhesion. Unlike in hemophilia, however, they do not develop hemarthrosis. They may have prolonged bleeding time and activated partial thromboplastin time (aPPT) depending on the Factor VIII level, but the most sensitive and specific diagnostic lab test is the platelet function assay [65].
Milder forms of hemophilia A and vWD often do not require factor replacement as they can be treated with desmopressin in older children and adults. Desmopressin (DDAVP) is a synthetic analogue of vasopressin [96]. A dose of 0.3 μg kg–1 IV increases Factor VIII:vWF two-fold to three-fold in 30–60 minutes [97]. When DDAVP is ineffective, as in vWD type 2 in which it may increase abnormal vWF or worsen thrombocytopenia, Factor VIII:vWF concentrates such as Humate-P are used [92]. DDAVP is also not given to young children and infants because of the risk of free water retention, hyponatremia, and seizures. Finally, in the perioperative period, consultation with a child’s hematologist is important in establishing the appropriate dose of DDAVP or Humate-P.
Complications Associated With Transfusion
Infection
When seeking consent from patients and their families for product transfusion, providers learn that there is perhaps no more feared complication than the risk of infection – particularly the risk of infection with HIV. In spite of the patient concern, however, the risk of acquiring a major viral infection from a transfusion is extremely low. Donor-screening, eliminating the use of products from self-identified high-risk donors, and viral testing of products has ensured that the incidence of viral transmission is now so low as to be almost nonexistent (Table 15.5).
Table 15.5 Viral infection risk
Disease | Incidence with transfusion |
---|---|
Hepatitis B virus | 1 in 137 000 |
Hepatitis C virus | <1 in 1 000 000 |
Human immunodeficiency virus | <1 in 1 900 000 |
Human T lymphotropic virus types I and II | 1 in 250 000 to 1 in 2 000 000 |
As viral infectious risk has fallen, the risk of bacterial infections has gained attention. Of the 694 transfusion-related deaths between 1985 and 1999, 11.1 percent were due to bacterial contamination [65]. Yersinia enterocolitica is the most prevalent contaminant of stored red blood cells [98] and the risk of contamination is directly related to length of storage [65,99].
Because platelets are stored at room temperature, the risk of bacterial growth in these products is higher and their shelf life is only five days. There is also evidence of greater infectious risk when pooled donors are used than when an apheresed unit from a single donor is given. Staphylococcus aureus, Klebsiella pneumoniae, Serratia marcescens, and Staphylococcus epidermidis are among the most common causes of death from bacterial infection following platelet transfusion. The mortality rate from platelet-related bacterial infection is 26 percent [100].
Hyperkalemia and Hypocalcemia
During storage and with aging, red blood cells leak potassium into the extracellular fluid. Although there does not seem to be clinically significant hyperkalemia when packed red blood cells are given slowly through peripheral IV access [65], the Perioperative Cardiac Arrest Registry reported eight hyperkalemic cardiac arrests related to blood transfusion [101]. With the rapid administration of large volumes of whole-blood or packed red blood cells, especially through a central venous catheter, hyperkalemia can develop [102]. This may be more likely to occur in infants and small children in whom it is easy to transfuse products at a rate >1.5–2 ml kg–1 min–1. Therefore, it is especially important to be vigilant to ongoing intraoperative blood loss, avoiding situations in which massive, rapid transfusion of products is necessary.
When products do need to be given, they should be warmed and infused through peripheral intravenous access. When massive transfusion is necessary, newer products – those that have spent less time in storage leaking potassium – should be used whenever possible. Further, if fresh products are not available, to the extent that time permits, washing the RBCs can be done to reduce the extracellular potassium. Hyperkalemia is also worse in units that have been irradiated. As such, these units can be stored for less time and need to be washed prior to administration to very young patients. Finally, when the infusion rate is greater than 1.5–2 ml kg–1 min–1, the ECG should be monitored for peaked T waves and treatment for hyperkalemia – calcium, sodium bicarbonate, albuterol, insulin, and glucose – should be readily available.
Stored blood components are anticoagulated with citrate, which chelates ionized calcium. Upon transfusion, the citrate is taken up by all cells but cleared primarily in the liver. In cases of massive transfusion, especially with whole-blood or FFP, citrate is transfused faster than the body can clear it, causing a decrease in the concentration of ionized calcium [103,104]. The ionized calcium falls enough to decrease cardiac contractility when the citrated product is transfused faster than 1.5–2 ml kg–1 min–1 [65]. When citrated products do need to be delivered at a rapid rate, exogenous calcium should be administered. Neonates and small infants may not eliminate citrate as rapidly, putting them at particular risk for hypocalcemia. Also, owing to its clearance in the liver, citrate toxicity may develop more readily in patients with hepatic dysfunction and during the anhepatic phase of liver transplantation [105].
Transfusion-Related Acute Lung Injury
The leading cause of transfusion-related death in the United States is transfusion-related acute lung injury (TRALI) [106]. The estimated risk of TRALI is 1 in 5000 transfusions, and it is most commonly associated with FFP [65]. TRALI is thought to be caused by an interaction between antibodies from the donor interacting with leukocyte antigens in the recipient, resulting in neutrophil activation in the pulmonary vascular bed. Clinically, its symptoms resemble acute respiratory distress syndrome – dyspnea, hypoxia, noncardiogenic pulmonary edema – and typically begin 4–6 hours after transfusion. Supportive therapy is indicated and 90 percent of patients recover [64].
Monitoring Intravascular Volume Status
Because ongoing losses, including blood loss, may be especially significant in the neonate or infant, estimation of intravascular volume status is difficult. Blood loss estimation is made quite difficult because small amounts are difficult to quantify and these small amounts may be significant, given low body weights of the patients. There has even been some interest in the use of cameras to detect and estimate blood loss. However, invasive monitoring of volume status, estimates of fluid losses, and clinician vigilance are the tools most readily available in the operating arena at this time and those monitors most frequently used in infants and neonates will be discussed here.
Central venous pressure (CVP) has been a longaccepted standard for the monitoring of intravascular volume status. The physiologic basis for this monitor is that superior vena cava (SVC) pressures approximate right atrial pressures (RAP), which reflect right ventricular preload [107]. However, placement of a central venous catheter (CVC) carries risks including arterial puncture, pneumothorax, and infection [108]. Patient factors become very important when deciding the site of CVC placement. It may be very difficult to place a subclavian line in a very small patient using anatomical landmarks. Ultrasound can be used to facilitate CVC placement and may reduce complications associated with insertion [109].
Peripherally inserted central catheters (PICCs) can also be easily inserted for monitoring and access purposes. Their advantage over traditional central lines is a low or similar infection rate, decreased complications of placement, and decreased need for general anesthesia to facilitate placement [110]. While the course it takes during placement is less predictable, this can be overcome with use of fluoroscopy. PICC lines can also provide another reliable route to monitoring of CVP.
Traditionally, the SVC was the most trusted site for CVP monitoring, but some alternatives have been studied. Measuring CVP in the inferior vena cava (IVC) has been shown to correlate well with RAP as measured in infants and children in the cardiac catheterization laboratory [111]. Peripheral venous pressure (PVP) has been studied and correlates well with CVP in infants, children, and adults [112]. PVP has also been shown to correlate well with CVP in infants and children with congenital heart disease and in children undergoing major surgical procedures [113,114]. However, neither bedside ultrasonographic measurement of the IVC to abdominal aorta (AA) ratio nor the IVC collapsibility index correlated well with CVP in acutely ill children [115].
Use of CVP as a monitor for intravascular volume status has been called into question by several studies. Specific concerns with CVP include its susceptibility to problems with venous return, right ventricular compliance, left ventricular failure, peripheral venous tone, patient position, valvular heart disease, increased abdominal pressure, and pulmonary vascular disease. Trends are likely to be more useful for interpretation than discrete measurements of CVP [116].
Transpulmonary indicator dilution (TID) has been advocated in neonates and infants, though there have not been large trials regarding outcomes. Transpulmonary indicator dilution has been validated in infants and children as an accurate measurement of cardiac output and systemic vascular resistance (SVR) [117]. Transpulmonary indicator dilution has been shown to more accurately indicate changes in intravascular volume status than CVP [118]. Many of the commercially available TID devices also utilize arterial pulse contour analysis to provide continuous cardiac output monitoring. Measurement of cardiac output and mixed venous oxygen saturation by pulmonary artery (PA) catheterization is rare in neonates and infants due to the high associated complication rate [119].
Arterial pulse pressure variation (PPV) and systolic pressure variation (SPV) are other common methods to estimate changes in intravascular volume status. More specifically, PPV and SPV are useful measures of fluid responsiveness. The principle behind these methods is that greater arterial pulse pressure changes with positive pressure ventilation will occur because of a greater drop in right ventricular preload, a greater increase in right ventricular afterload, and a decrease in left ventricular afterload [120]. In cases where wide swings in intravascular volume status are anticipated, an arterial line is often placed. Since these methods require only an arterial pressure waveform for analysis, it is a valuable tool that adds little to the required monitors or equipment for the procedure. Its reliability is decreased in the setting of spontaneous respiration, arrhythmias, pulmonary hypertension, right heart failure, low tidal volume, and open chest [121]. Systolic pressure variation greater than 10 mmHg is indicative of a fluid responsive state [122].
Urine output is a frequently utilized monitor for intravascular volume status. Despite renal system immaturity, the neonate and infant will restrict urine output in response to decreases in intravascular volume. Oliguria, less than 1 ml kg–1 h–1 in infants and 0.5 ml kg–1 h–1 in children, is often, but not always, indicative of hypovolemia [123]. Confounding factors include other hypotensive states that decrease GFR, SIADH, intrinsic renal failure, and mechanical problems with urinary catheters. However, in the presence of other physical signs, oliguria can be a useful monitor of intravascular volume status.
Echocardiography is a reasonable monitor in the intraoperative setting. With a variety of probe sizes available, even very small neonates can be monitored safely with transesophageal echocardiography. If the chest is unobscured by the surgery, transthoracic echocardiography may also be used, but not continuously. Inferior vena cava measurement, SVC collapsability, ventricular filling, systolic left ventricular cavity obliteration, and left ventricular end-diastolic area have all been described as methods for diagnosing hypovolemia [124].
Vascular Access Strategies
Vascular access can be challenging in neonates and infants. The process of obtaining access is challenging and the management of that access remains difficult. To further complicate matters, many neonates and infants who present for surgery have had multiple prior access sites.
Traditional peripheral intravenous (PIV) access is the most common technique encountered. When vasculature cannot be easily located visually or by palpation, there are several technologies to aid in vein localization. Anatomy-based techniques are popular, where PIV access is attempted without visualization, palpation, or the aid of a medical device. Common sites to attempt this method of PIV cannulation are the great saphenous vein, the cephalic vein (“intern’s” vein), veins on the dorsum of the hand, and antecubital venous access because of their more consistent anatomy.
The external jugular vein is frequently utilized in a difficult access situation. However, the vein is quite mobile and carries the additional risk of pneumothorax or carotid cannulation. The mobility of the site also results frequently in catheter dislodgement. Scalp veins are also utilized in this age group, whereas they are frequently not utilized in older children or adults. Scalp veins are also prone to dislodgement, infiltrations are not inconsequential, and temporal artery cannulation is possible. Surgical cut-down for peripheral venous access is another possibility, but it is difficult, time-consuming, and associated with increased patient morbidity.
Several technologies have been made available for PIV cannulation in neonates and infants. Ultrasound is a frequently used technology and has been studied versus the anatomical-based approach to difficult PIV access. It was found that both techniques are effective in venous cannulation, but ultrasound was faster and more likely to be successful on the first attempt than the “blind” technique [125]. Transillumination is another technique useful in neonates and infants for PIV placement [126]. Local warming of proposed cannulation sites and epidermal nitroglycerin have been used to increase success of percutaneous PIV insertion [127,128]. Near-infrared (NIR) vein scanners are available in several different commercial variants. They emit NIR light and reveal a vein map because the light is scattered by forward flow of blood. The light scatter is detected by the device and a vein map is projected on the patient to facilitate PIV insertion [129].
Central venous access is frequently utilized in neonates and infants without incidence. One of the most common methods of central venous access in neonates are umbilical venous catheters (UVCs). Traditionally, the biggest risks have been malposition and infection, but one study has advocated that long-term use of UVCs does not necessarily increase risk of infection over replacing the UVC with a PICC [130]. The umbilical vein is 2–3 centimeters long and then joins the left branch of the portal vein. At this juncture, the ductus venosus arises, which bypasses the liver and joins the IVC just distal to its entry into the atrium. The ideal position for the UVC is in the IVC, but in emergency situations the catheter can be placed shallowly in the umbilical vein, though care must be taken to avoid hyperosmolar solutions which can cause liver necrosis. Umbilical arterial catheters (UACs) can also be placed. The umbilical arteries are a direct continuation of the internal iliac arteries and thus a UAC will pass through the umbilical artery to the internal iliac artery, then go behind the bladder to reach the aorta. The UAC should be placed high enough within the aorta (above the diaphragm) to avoid inadvertently occluding the celiac axis, renal arteries, or superior mesenteric arteries. PICC lines can be placed in even the smallest of neonates, frequently using the femoral site for cannulation. Midline catheters are similarly inserted in the deep veins of the upper arm, but terminate outside the thorax, as opposed to centrally.
Traditional sites for CVC placement are the same as in older children and adults: femoral, subclavian, and internal jugular. While the external jugular is sometimes used to place a CVC, difficulty is often encountered in navigating the catheter into the central circulation [131]. Adult studies have generally shown the subclavian site to have the lowest risk of infection, but in children it has been identified that the more important risk factors for infection are younger age and duration of CVC use [132]. Complications related to insertion are highest with the subclavian site and complications related to maintaining the central line were more common in younger children and in the femoral site of access [133] [134]. A systematic review of central line literature suggested that implanted ports may be more often recommended in children than in adults for longer periods of use, and that central line placement be routinely confirmed with radiograph given the high risk of malposition in children [135].
Intraosseous (IO) lines are frequently used in critically ill neonates and infants, and are safe to place [136,137]. They should be considered when other routes of intravenous access have been unsuccessfully attempted. In neonatal emergencies, they are faster to place than a UVC [138]. There are reports of IO lines being successfully placed and used in the perioperative period [139]. However, IO lines are not without complications, which include fat embolism, infection, fractures, and malposition or dislodgement. Malposition or dislodgement can result in a compartment syndrome [140]. Intraoperative dislodgement would be especially concerning, given the ongoing difficulty in vascular access at this point, with very little access to the patient under surgical drapes. While the decision to employ an IO line should be approached with caution, this important route to vascular access should be considered.
References












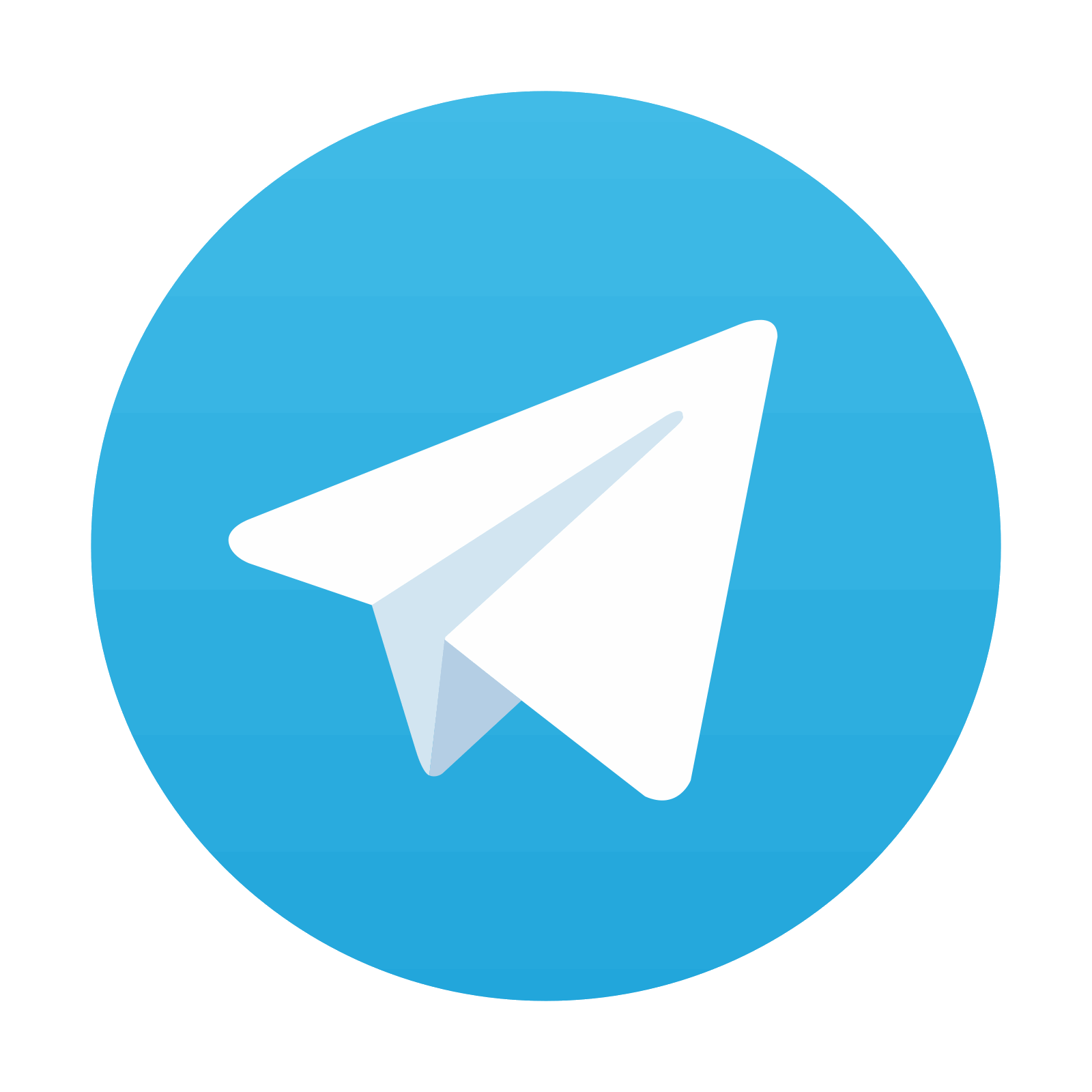
Stay updated, free articles. Join our Telegram channel
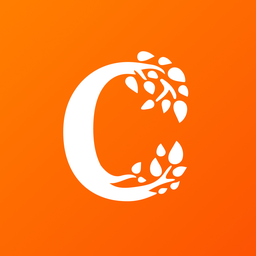
Full access? Get Clinical Tree
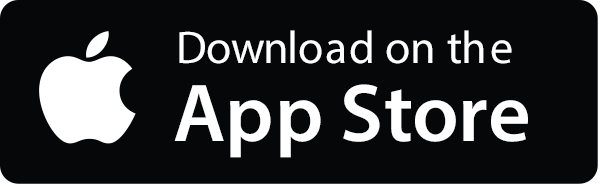
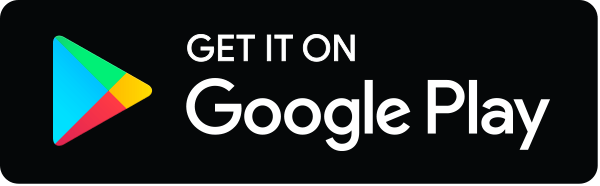
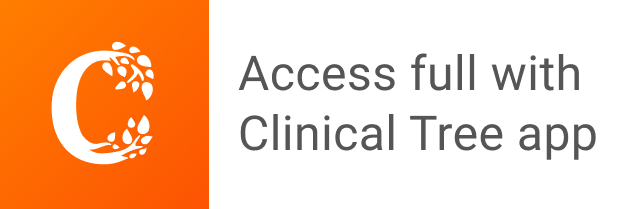