Neonatal and Pediatric Pharmacology: The Evolution of Scaled Modeling
In 1950, Terry et al. boldly stated what practicing pediatricians had known clinically for so long – neither age nor weight were satisfactory metrics for drug calculations in children. They therefore proposed scaling drug dosages according to body surface area, and were able to support this hypothesis with empirical evidence [1]. This scaling calculation was extended to water, electrolytes, and calories, preceding Holliday and Segar’s guidance for parenteral fluid therapy according to basal metabolic rate by seven years [2]. Allometric (nonlinear, growth-based) scaling entered the mainstream of dosing concepts at that time, but was embraced by relatively few, and rather simplistic pharmacology models persisted for decades (Figure 13.1).
Figure 13.1 A simplistic – and common – concept map of dosing and elimination.
Pediatric pharmacokinetic parameters are now understood with increasing precision. Age-adjusted volume of distribution is important for the loading dose and of crucial importance in anesthetic practice because of the need to rapidly achieve a target effect. Clearance determines the maintenance dose rate. Together, clearance and volume of distribution determine the shape of the concentration–time curve [3]. Pharmacodynamic parameters, particularly for children and more so for neonates, are a far different story. Almost all neonatal organ systems are still developing; some enzymes are present, some are absent, some are increasing in activity, some are decreasing. Hematocrit affects drug delivery, as does the concentration of proteins (and the types of proteins) in the blood. Many organ systems have a relatively larger mass within a neonates body – does that mean they function as hypercellular systems, or are those systems less mature despite the increase in mass? Effective drug delivery to the receptor/effector sites depends on blood flow, tissue volume, tissue solubility coefficients, and protein binding. Babies are different in almost all of these respects than children and adults.
Effective Modeling: Why are Children Different?
Volume of Distribution
Because a child’s job is growth and development, he or she is obligated to a ravenous early life of oxygen consumption, acquisition of calories, and production of heat. In order to survive as homeotherms – without burning up – they must have a way to exquisitely balance heat generation and heat loss, which led to the proposal of the Surface Law (homeotherms maintain their temperature homeostasis in proportion to their body surface area, i.e., heat production = heat loss) [4]. This turned out to be almost right. When oxygen consumption is measured directly or indirectly, it is actually more proportional to body mass (kg)3/4 (Figure 13.2), whereas body surface area is calculated as (kg)2/3 [5]. It turns out that thermal homeostasis is proportional to the vascular branching necessary to deliver heat to the skin surface, rather than the skin surface itself. The capacitance of this branched system, influenced by the scaling concept, formed the basis for Holliday and Segar’s fluid recommendations [2].
Figure 13.2 A log-linear plot of basal metabolism of mature animals of different species, with an almost uniform slope of 0.75 – the ¾ power, if expressed exponentially. The slope of the line is the exponent of the function. When the exponent = 1, there is isometry (isometric growth), meaning there is no change in shape during growth.
Accurate anticipation of differences in the volume of distribution is of crucial importance in anesthesiology in order to rapidly achieve a target effect. With a naturally larger volume of distribution because of allometric scaling as well as a larger “effective” volume of distribution because of a decrease in plasma proteins, a larger (apparent) volume of distribution is the result. This may explain the rather ironic requirement for a larger initial drug dose in infants and small children in order to achieve target concentration parity with adults and even older children (Figure 13.3).
Figure 13.3 Enhanced clearance and a larger central compartment volume results in reduced blood concentrations compared with adults. Smaller children require higher infusion rates (weight-based) than larger children or adults to achieve equivalent blood concentrations.
Clearance
Scalar “per kilogram” models of clearance under-predict, while surface area models over-predict clearance, leading to errors in calculating clearance with decreasing weight. Scalar modeling, for example, will lead to the false conclusion that children possess an enhanced capacity to metabolize drugs due to proportionally larger livers and kidneys (more “cellularity” per kilogram of body weight) than in adults [7]. As a better alternative, allometry is a good method of scaling clearance when only size differences exist [3]; however, it will overestimate clearance in children when metabolic clearance pathways are developmentally immature (e.g., activity of the enzyme per unit volume of tissue is lower). This method should only be used alone when the patient’s clearance for age reaches similar activity (>2 years) to that of adults [8]. A maturation model is required to describe clearance in neonates and infants (<2 years) to account for changes independent of size.
Cardiac Output
One consequence of the allometrically scaled increased cardiac output and organ size in infants (i.e., the brain receives 30 percent compared to 15 percent of this proportion-adjusted cardiac output) is that more drug reaches target receptors more rapidly in vessel-rich organ groups (brain, kidney, intestinal viscera, especially the liver).
Renal Clearance
Excretion may be reduced compared with older children and adults because glomerular and tubular renal function are reduced in newborns. Using an allometrically scaled postmenstrual age (gestational age + chronological age = PMA) model, Rhodin et al. reported adult GFR as 121.2 ml min–1 per 70 kg, with half of the adult value reached at 47.7 postmenstrual weeks. At one-year postnatal age, the GFR is predicted to be 90 percent of the adult GFR [9] (Figure 13.4)
Figure 13.4 Maturation of GFR showing the predictions of the sigmoid hyperbolic function. The abscissa is expressed as weeks of postnatal age so that 0 would be a full-term infant with a postmenstrual age (PMA) of 40 weeks.
Hepatic Biotransformation
Hepatic biotransformation is unrelated to body weight and scaled growth. Some cytochrome P450 (CYP) enzymes are present at birth (CYP3A7, uridine 5′-diphospho-glucuronosyltransferase [UGT]), while others are not (CYP2E1, CYP2D6, CYP3A4, CYP2C9). Some achieve adult activity in a few days (UGT2B7), while some require months to years to mature (UGT1A6) [10] (Figure 13.5). Hepatic activity cannot be anticipated by weight, body surface area (BSA), nor allometric scaling [11].
Figure 13.5 Development and regression of cytochrome P450 (CYP) 3A4 and 3A7 as activity measured using isoform-specific probes in human liver microsomes.
The CYP system is immature at birth and does not reach adult functionality until the first or second month (Figure 13.6). This immaturity may explain the prolonged clearance or elimination of some drugs in the first few days to weeks of life, as well as the inability of the liver to convert a precursor into its active form. The CYP450 system can be induced by a variety of drugs (e.g., phenobarbital). The age from birth – not the gestational age – determines how premature or full-term infants metabolize drugs.
Figure 13.6 The activity of many cytochrome P450 (CYP) isoforms and a single glucuronosyltransferase (UGT) isoform is diminished during the first two months of life. The achievement of adult activity over time is enzyme- and isoform-specific.
Elimination may be further affected by abnormal or decreased liver blood flow as a result of illness or surgery. Certain neonatal conditions may increase intra-abdominal pressure, and also decrease liver blood flow (e.g., closure of an omphalocele or gastroschisis, reopening of a ductus venosus, positive pressure ventilation).
Binding
Opioids, amide local anesthetics, and muscle relaxants, for example, bind to albumin and alpha-1 acid glycoprotein. The unbound (free) drug is available to cross biological membranes, bind to receptors, and achieve a therapeutic concentration. Lower concentrations of albumin and alpha-1 acid glycoprotein result in more unbound drug availability to target receptors.
Organ Function
Pharmacodynamics are affected in a substantial way by anesthetic and critical care interventions. For example, positive pressure ventilation may be associated with reduced clearance. This effect may be attributable to reduced hepatic blood flow with a drug that has perfusion-limited clearance (e.g., propofol, morphine) [3].
Pharmacodynamics: Developing Organ Systems and Drug Targeting
Most investigations center on pharmacokinetics (PK), but dosing predictions as well as monitoring for therapeutic and adverse effects also requires a better understanding of pharmacodynamics. The challenge in caring for infants is that receptors themselves are in a state of development and may therefore express themselves with much greater variability quantitatively and qualitatively [7]. For example, neuroplasticity is characteristic of the developing nervous system centrally and peripherally, and there is no reason to think that it is not integral to developmental nociception. Maturation considerations for neurochemistry and neuroanatomy may alter drug efficacy in the neonate. While anatomic and neurotransmitter mechanisms are present at birth for the processing of painful stimuli, these systems evolve through the fetal and newborn period and into adult life, and it is not likely that they function in an identical manner during these lifecycle changes. Glutamine and substance P (SP) are colocalized in C fiber terminals in the dorsal horn and facilitate pain transmission in part by activating N-methyl-D-aspartate (NMDA) receptors. In the adult rat, NMDA receptor binding is restricted to the substantia gelatinosa (SG), but at postnatal day 7 (P7) in rat models it is distributed throughout the spinal cord; the more mature and discretely distributed pattern is not achieved until P28 and parallels SP and other neuropeptides (Figure 13.7). In the rat, SP receptors move from a diffuse, nonspecific distribution in the neonate to discrete and concentrated loci in the mature SG. These developmental considerations in the expression of SP as well as other neurotransmitter systems make the neonatal SG substantially different than that found in adults, notwithstanding the achievement of calculated adequate drug levels systemically or for regional anesthetics.
Figure 13.7 Mu receptor binding sites at P1, P7, P14, and P28 and at different levels of the adult rat spinal cord. In human terms, these ages are approximately 1.5 m, 19 m, 3.3 y, and 7 y.
Cardiac effects of many anesthetic drugs are not the same in neonates because the neonatal heart is different than the adult heart, or even the heart of an older child. Neonates have a cardiac output 2–3 times that of adults, about 180–240 ml kg–1 min–1. Cardiac muscle mass is smaller in newborns and infants than in older children and adults, the ventricles are less compliant, and in addition, there is a higher resting tone at end-diastole, with a lower peak pressure achieved during systole. There is also a developmental basis for differences in excitation–contraction coupling. Tension development in the myocardium is activated by the influx of Ca2+ across the sarcolemma. As the myocardium matures, intracellular Ca2+ uptake and re-release by the sarcoplasmic reticulum plays an increasingly important role in tension development [14]. Calculations for drug dosing in neonates must take into account that neonatal myocardial depression may be more than just a dosing effect, but rather an adverse effect in the context of a substantially dissimilar myocardium.
Population Pharmacokinetics and Neonatal Variability
Classical pharmacokinetic studies involve administering single or multiple doses of a drug to a small group of subjects and then obtaining multiple samples to describe the concentration and time profile of the drug. The highly charged ethical and practical issues of conducting such studies in children, especially neonates, are obvious. A preferable approach in most pediatric situations is the population pharmacokinetics (PPK) approach, combining fewer samples of individual data with blood sampling from a large target population at varying times.
Population pharmacokinetics is focused on absorption, distribution, metabolism, and excretion, using mathematical and statistical modeling, typically nonlinear mixed effects modeling (NONMEM). For example, using PPK and NONMEM modeling, Anderson et al. have shown that size explains 49.8 percent, age 18.2 percent, and renal function 14.1 percent of clearance variability of vancomycin in neonates [7].
Better Prediction Through Better Modeling?
In an attempt to incorporate the many aspects of pharmacokinetic pharmacodynamic modeling, particularly with regard to infants and small children, Simcyp® software (Simcyp Limited, Blades Enterprise Center, John Street, Sheffield S2 4SU, UK) integrates demographic, genetic, physiological, and pathological information on adults with in vitro data on human drug metabolism and transport to predict population distributions of drug clearance (CL) and the extent of metabolic drug–drug interactions (Figure 13.8). The algorithms have now been extended to pediatric populations by incorporating information on developmental physiology and the development/maturation of specific cytochrome P450s [15]. Simcyp® algorithms have been reported to provide reasonable estimates of the in vivo drug clearance of 11 drugs commonly used in neonates, infants, and children [16].
Figure 13.8 Simulated concentration–time profile for (a) midazolam alone (black line); (b) midazolam alone (black line) and in the presence of rifampicin (gray line); (c) midazolam alone (black line) and in the presence of rifampicin and fluconazole (gray line); and (d) midazolam alone (black line) and in the presence of rifampicin, fluconazole, and clarithromycin (gray line).
Inhalation Anesthetics
The effects of chloroform are more quickly produced and also subside more quickly in children than adults, owing no doubt to the quicker breathing and circulation [17].
So observed John Snow about inhaled anesthetics in children, providing early insight into fundamental differences and paving the way for pediatric anesthesiologists to be leaders in pediatric pharmacology.
Most of the PK differences are due to allometrically scaled parameters. Scaled branching and volume of the circulation is directly related to metabolic activity, since heat delivery from the core to the periphery has to be exquisitely balanced to maintain the homeothermic state. Developmental blood chemistry balance (differences in total proteins, albumin, cholesterol, and total body water) also affects tissue/blood solubility such that lower solubility is characteristic of neonates. These differences add to enhancing the speed of induction and the rapidity of therapeutic as well as adverse effects in children. These PK effects are outlined in Table 13.1.
Children | Adults | Implication | |
---|---|---|---|
Alveolar ventilation: FRC ratio | 5:1 | 1.5:1 | Enhanced wash-in |
Cardiac output | 20 dl min–1 (2 dl kg–1 min–1) | 50 dl min–1 (0.75 dl kg–1 min–1) | Shorter time to equilibrium and enhanced speed of induction |
Tissue:blood solubility | Neonates have lower levels of total protein, albumin, and cholesterol, and higher levels of total body water | ||
Total protein | 50 g L–1 | 70 g L–1 | Less protein available for binding therefore increased free fraction |
Albumin | 30 g L–1 | 37 g L–1 | Less protein available for binding therefore increased free fraction |
Cholesterol | 1.3 g L–1 | 2.09 g L–1 | Lower cholesterol, lower solubility, and increased free fraction |
Total body water | 689 g kg–1 | 605 g kg–1 | Greater dilution |
In addition to the differences in PK outlined above, anesthetic potency also influences the ability to rapidly adjust an inhaled anesthetic, whether during induction or any other phase. The minimum alveolar concentration (MAC) has been the standard used to express anesthetic potency, and in general the lower the MAC the more potent the anesthetic. Lipid solubility of the specific agent is inversely related to the MAC of that agent; in addition, MAC has long been recognized to be different for different age groups (Figure 13.9).
Figure 13.9 MACs of four commonly used inhaled anesthetics plotted vs. age. MAC is highest in infants 3–6 months of age.
Carbon monoxide (CO) can be produced in subclinical concentrations by all of the current methyl ethyl ethers (desflurane > isoflurane > halothane and sevoflurane) in the presence of desiccated soda lime or baralyme. Newer CO2 absorbents (those that contain a lithium-based catalyst) do not have the typical strong bases (KOH, NaOH) and do not result in carbon monoxide or Compound A production. Furthermore, they are not significantly exothermic. Mitigating effects in infants and children include a lower total minute CO2 production despite a higher per kilogram per minute value due to increased oxygen consumption.
Post-anesthesia delirium has been associated with all of the currently utilized inhalation agents, and there seem to be numerous cofactors that make it all the more difficult to discriminate a single etiology [19]. Again, this is a difficult assessment in the neonate and infant.
Specific adverse effects of inhalation agents more prominent in the neonatal and infant age group include:
Myocardial effects. Cardiac muscle mass is smaller in newborns and infants than in older children and adults, the ventricles are less compliant, and in addition there is a higher resting tone at end-diastole, with a lower peak pressure achieved during systole [20]. Conduction delays are almost uniformly predictable with halothane but are variable with the newer ethers.
Baroreceptor effects. Baroreception, like myocardial performance, “matures” postnatally. The sensitivity of carotid baroreceptors is reset as arterial pressure increases throughout the last third of gestation and the first postnatal month. Postnatally, baroreflex sensitivity increases from birth to six weeks of age with the most rapid changes occurring after two weeks of age [21–23]. Inhalation anesthetics may aggravate the relative insensitivity of the baroreceptor response in neonates [24,25].
Respiratory effects. All potent volatile agents depress minute ventilation and shift the CO2 response curve “up and to the left” in infants, more so than in older children and adults. Comorbidities such as the Chiara II malformation commonly associated with spina bifida worsen this finding.
Sevoflurane
Sevoflurane has become the most popular inhalation anesthetic for children because of its ease of acceptance, non-pungency, and relative forgiveness with regard to adverse cardiovascular effects in comparison with the previous generation’s gold standard, halothane [26]. After passing through soda lime or baralyme, sevoflurane is degraded into five compounds (Compounds A–E). Only Compound A accumulates significantly in the circuit when the following conditions are met: increased temperature, low water content, high concentration of sevoflurane, the use of baralyme rather than soda lime, or the use of new soda lime.
Sevoflurane has half the potency of isoflurane and one-third that of halothane. Interestingly, sevoflurane MAC does not increase with decreasing age, in contrast to the other inhaled agents. For unclear reasons, the additive effect of nitrous oxide is not as prominent with sevoflurane as it is with other agents.
There seem to be some unique electroencephalographic effects of sevoflurane, specifically epileptiform-like patterns, as well as rare facial or limb twitching during light levels that seem to disappear in deeper planes. The significance of these findings is also unclear and no adverse outcomes have been linked to these findings.
Desflurane
Desflurane is isoflurane with replacement of the chlorine with a fluorine – but what a difference pharmacologically! The blood–gas and tissue–blood solubilities are dramatically decreased and consequently the uptake and distribution as well as the washout of desflurane is the most rapid of any of the potent inhaled anesthetics. Unfortunately, the pungency and airway irritability of desflurane make it a poor clinical choice for inhalation inductions in children, but maintenance and emergence are not problematic following an intravenous induction or an inhalation induction with a less pungent (i.e., sevoflurane) anesthetic.
Emergence, as expected, is quite rapid because of the rapid washout, and therefore a plan for preemptive postoperative analgesia should be coupled with the use of desflurane as the principal anesthetic. There would appear to be little need for utilizing desflurane clinically in neonates and infants for the sake of facilitating rapid emergence or even rapid intraoperative adjustments in anesthetic depth because of their relatively small tissue stores and rapidity of equilibration with changes in the inspired agent concentration.
Isoflurane
The wash-in and wash-out of isoflurane is slower than desflurane or sevoflurane. Like desflurane, it has a pungent odor, making it less favorable for mask induction in children; maintenance and emergence do not present significant clinical issues, the pungency notwithstanding. Infants anesthetized with isoflurane do not exhibit the same degree of reduction in heart rate that results from halothane use.
Nitrous Oxide
Nitrous oxide has a long history of use in infants and small children. Its low blood–gas partition coefficient and low potency have two very appealing features for use in infants – it works rapidly and because of its lower potency may actually provide a greater margin of safety for infants. As a carrier gas it has featured prominently throughout the history of pediatric anesthesia because of the second gas effect.
Caution must be applied with regard to its tendency to occupy air-filled spaces due to its blood–gas partition coefficient being 34-fold greater than nitrogen. Inasmuch as bowel obstruction is one of the more common newborn surgical emergencies, clinicians must be circumspect about the use of nitrous oxide. More subtle considerations include its effect on the inhibition of methionine synthetase, an important consideration for infants with their developing bone marrow and nervous systems.
Intravenous Induction Agents
Barbiturates have long been the standard intravenous induction agent for all age groups. Special pediatric considerations over the years have been recognized – for example, prolongation of sleep times of newborns (presumably due to greater blood–brain barrier permeability), impaired biotransformation due to glucuronic acid conjugation immaturity in the first several weeks of life, and an increased dosing requirement in the newborn period and especially for the first six months of life.
Thiopental is a highly lipid-soluble ultra-short-acting barbiturate that results in rapid unconsciousness. Plasma protein binding is reduced in the neonate, resulting in a doubling of the plasma-free fraction in comparison to children and adults. In addition, clearance is substantially reduced in infants (less than half that of adults). Because its effect is primarily terminated by redistribution, recovery in infants nevertheless is on par with older children and adults. Thiopental has not been available in the United States since 2011.
Methohexital, a more potent barbiturate than thiopental, has not commonly been used by the intravenous route in children. It has mainly been used rectally, and a body of literature has described blood levels and reliability as an induction agent in infants and older children, but not in neonates.
Virtually all intravenous inductions, when chosen, are currently accomplished with propofol. Its short duration of action because of rapid redistribution and biotransformation permit its use by bolus as well as continuous infusion. Glucuronidation is the major route of elimination. Uridine 5-diphosphate-glucuronosyltransferases (UGTs) occur at varying times during early infancy, and some UGTs are present at birth while others develop during early infancy. A decrease in total requirements may be due to a net immaturity of neonatal enzyme systems while a decrease in induction dose requirements probably has more to do with a greater tendency toward hypotension and bradycardia in infants [27]. Propofol may also limit its own biotransformation by virtue of its effect on cardiac output and hepatic blood flow in neonates and infants, although few studies exist describing neonatal propofol pharmacology. Other features noted in patients who can self-report them – pain on injection, antiemetic effects – may occur in infants as well, but are probably more difficult to detect.
Ketamine remains a significantly utilized alternative to propofol because it preserves cardiovascular stability and rapidly results in unconsciousness. Infants typically require a much larger dose to produce lack of movement with induction than older children. In infants younger than three months, the volume of distribution is similar to older infants, but the elimination half-life is prolonged. Clearance is reduced in younger infants with reduced metabolism; slower renal excretion is the likely cause. While respiratory efforts tend to be preserved in children and adults, respiratory depression and apnea may be the result of higher doses required in infants. Muscular (extensor) spasm may also occur, as well as elevations in pulmonary artery pressure in infants with an underlying elevation in baseline pulmonary artery pressure (congenital heart disease).
Sedatives
Benzodiazepines
Benzodiazepines are commonly used in pediatric patients to provide sedation, anxiolysis, anterograde amnesia, as well as anticonvulsant therapy. Benzodiazepines bind to gamma amino butyric acid (GABA) receptors, which are distributed throughout the frontal cortex and cerebellum in varying densities during the fetal through neonatal period [28]. Metabolism occurs in the liver by cytochrome P450 enzymes with excretion in the urine. The clearance of these drugs varies depending on clearance of the parent drug and its metabolites, as well as the intrinsic physiology of neonates and their comorbidities.
Midazolam is lipid-soluble at physiological pH and therefore has a rapid onset compared to other benzodiazepines. It also has a shorter half-life compared to diazepam and lorazepam. Absorption via the gastrointestinal tract is slower in premature neonates, full-term neonates, and young infants secondary to delayed gastric emptying [12,29]. Rectally administered midazolam may have faster absorption, but the bioavailability may vary.
Midazolam is converted to the active metabolite 1-hydroxymidazolam (1-OH-midazolam) by cytochrome P450 enzymes CYP3A4, CYP3A5, and, to a lesser extent, CYP3A7 [30,31]. 1-OH-midazolam has a shorter half-life compared to other benzodiazepine metabolites, yet in neonates the half-life can be anywhere from four to six hours, compared to two hours in adults [30,31]. CYP3A4 and CYP3A5 are activated during the first week of life [29] and, therefore, the formation of 1-OH-midazolam is decreased and clearance of midazolam is slower in preterm and full-term neonates [31,32].
Diazepam is a longer-acting benzodiazepine commonly administered via oral, intravenous, or rectal routes. It is also metabolized by cytochrome P450 enzymes into desmethyldiazepam, which has a longer half-life in premature and term neonates compared to older children [28]. While highly bound to plasma proteins, the amount of unbound drug in newborns is double that of adults, so there is a potential for accumulation and a prolonged drug effect. The adult ratio of bound:unbound drug is reached by the first week of life.
Lorazepam is usually administered intravenously to the neonatal population. While some PK data exist for intramuscular and submucosal routes in the healthy adult population [33], there is still a lack of data in neonates and infants. It is a long-acting benzodiazepine, with half-life ranging from 18 to 73 hours in neonates, compared with 10–12 hours in older children and adults [34]. While also metabolized by cytochrome P450 enzymes, its metabolite is inactive. Lorazepam is about 75 percent plasma protein bound and also excreted in urine.
Any comorbidities that cause changes in hemodynamic, renal, or hepatic function or concomitant medication administration may also alter metabolism and excretion of benzodiazepines, leading to accumulation and prolonged sedation. Burtin et al. showed decreased clearance by 30 percent in neonates receiving sympathomimetic drugs, which the authors attributed to compromised cardiac output in critically ill neonates [32]. De Wildt et al. showed newborns exposed to indomethacin for treatment of patent ductus arteriosus (PDA) had higher clearance of midazolam compared to those who were not exposed; however, a decrease in renal function with the use of medications such as indomethacin and NSAIDs should also be considered in the neonate receiving benzodiazepines [31].
Interindividual variability in expression and activity of the CYP enzymes may also account for the interindividual variability in the clearance of benzodiazepines in neonates [28,29,30,32]. Exact neonatal dosing for a target concentration is difficult. Moreover, drugs like midazolam, which is considered short-acting in adults, may not have the same clinical profile in neonates. Benzodiazepines should therefore be administered and titrated to clinical effect, with the potential for accumulation in mind.
Dexmedetomidine
Dexmedetomidine is a selective α2-agonist increasingly used in pediatric anesthesia for sedation or as an adjunct [35–39]. It has an α2:α1 activity of 1620:1, compared to clonidine’s 200:1, and acts on the locus ceruleus to provide sedation and analgesia. Dexmedetomidine is metabolized in the liver by glucuronidation, hydroxylation, and N-methylation. It is about 90 percent protein-bound to albumin, and is eliminated primarily in the urine. In adults, dexmedetomidine has a half-life of about two hours, compared to clonidine’s half-life of 12–24 hours. In newborns, the half-life is about 7.6 hours in preterm neonates and 3.2 hours in term neonates [40]. Using PPK, Potts et al. found clearance in neonates in the ICU to be about one-third that of adults. Clearance reaches about 87 percent of adult values by one year of age [41]. All of these findings are attributed to immature metabolic and elimination pathways in the neonate.
The hemodynamic profile of dexmedetomidine has also been widely studied in adults and children [42–46]. Its known effects on heart rate, and blood pressure, have also been seen in neonates [39,47]. While its safety profile continues to be studied and its use generally accepted among the pediatric population, it’s PK profile in the neonate may warrant lower dosages to avoid unwanted effects [40].
Opioids
Opioids are commonly used in neonates for analgesia and sedation. Similar to benzodiazepines, several studies have shown age to be the most important variable that affects metabolite formation, morphine clearance, and plasma concentration [48,49]. In neonates and infants, the beta elimination half-life of opioids is prolonged compared to adults and older children, so respiratory depression may also be prolonged. Opioids are primarily metabolized in the liver via oxidation, except morphine which undergoes glucuronidation and remifentanil which clears via ester hydrolysis [50]. Most opioids are also protein-bound to albumin and α1-acid glycoprotein, both of which are decreased in the neonate and, therefore, cause increased concentrations of unbound drug. Excretion is mainly via the kidneys.
Morphine is metabolized into morphine-3-glucoronide (M3G), which has no analgesic properties, and morphine-6-glucoronide (M6G), the active metabolite. The plasma concentration ratio of M6G:morphine is higher after oral administration than after intravenous administration due to the first-pass mechanism [50]. Among neonates and infants, the M3G metabolite also predominates [49]. Since there is less of the active metabolite, M6G, there may be a need for a higher concentration for an analgesic effect compared to older children and adults. As a result, there may also be more undesirable effects of the opioid, such as hypotension, bradycardia, hypercarbia, and seizures. Using a PPK approach, Bouwmeester et al. found that higher morphine infusion rates were necessary for infants and young children to achieve a target plasma concentration of 10 ng mL–1, with the highest rate required at one year of age. Total body clearance of morphine reached 80 percent that of adults by age six months [49]. Once M3G and M6G metabolites are formed, they are excreted via the kidneys. Excretion then becomes a function of GFR. Because neonates have decreased glomerular and tubular function, the rate of elimination will also be slower and lead to accumulation of active metabolites.
Fentanyl is highly lipid-soluble and 50–100 times more potent than morphine, with rapid onset and shorter duration. It is also known to be relatively cardiac-stable and used widely in cardiac anesthesia as well as for sedation in the NICU. Fentanyl is metabolized in the liver by CYP3A4 enzymes via N-dealkylation and hydrozylation into inactive metabolites, and excreted in the urine. It is mostly bound to albumin, and less so to α1-acid glycoprotein. In adults, metabolism of fentanyl is not significantly altered with liver cirrhosis, but may be altered with impaired hepatic blood flow [50]. Transmucosal and transdermal routes of administration have been used and studied in older children [48,49], but their use and pharmacokinetic profile has not been delineated in neonates.
Remifentanil is a synthetic opioid with a potency similar to that of fentanyl. It is fast-acting and has a very short half-life. In adults, the beta elimination half-life is 9.5 min, while studies in neonates show it to be 3.4–5.7 min [51,52]. It is unique compared to other opioids because it undergoes ester hydrolysis in the plasma. The clearance rate in neonates is about twice as long as adults; however, liver disease, kidney disease, or the use of cardiopulmonary bypass do not appear to affect the clearance of remifentanil [51,53].
Sufentanil is also a highly lipid-soluble, synthetic opioid 5–10 times more potent than fentanyl. Like fentanyl, it has been shown to be appropriate for use in pediatric cardiac patients because it maintains relative hemodynamic stability. Sufentanil is metabolized by N-dealkylation into inactive metabolites and excreted in the urine [50]. It is mostly bound to α1-acid glycoprotein [54,55]; thus, more active, unbound drug can be found in the plasma of neonates. Moreover, protein binding is affected by plasma pH such that alkalosis would decrease and acidosis increase binding [55].
Alfentanil is another fast-acting opioid with a short half-life (5–10 min). It is one-third less potent than fentanyl and bound mainly to α1-acid glycoprotein. Unlike sufentanil, plasma pH does not affect its protein binding [50]. Alfentanil is metabolized by CYP3A4 and excreted in the urine. Like fentanyl, muscle rigidity has been found to occur in neonates receiving alfentanil infusions [56].
Acetaminophen
Acetaminophen is a commonly used over-the-counter drug to treat fever and pain. It is water-soluble and therefore has potentially a lower peak concentration in neonates, given their higher volume of distribution. This would lead to less effectiveness and the need for a higher loading dose. While maturation may account for about one-third of the variability in absorption, other factors that can contribute to slower absorption in the neonate include their slower gastric emptying, and concomitant administration of food or other medications [57]. Absorption after rectal administration is much faster compared to oral administration, but the bioavailability of the drug may also have interindividual variability, depending on where in the rectum it is delivered. Although metabolism is by glucuronidation in a mature system, acetaminophen undergoes primarily sulfate conjugation and renal excretion in the neonate. Thus, one can expect slower clearance and the potential for accumulation and toxicity. Adult clearance is usually reached by 12 years of age [58].
Muscle Relaxants
Neuromuscular blockers (NMBs) are used intraoperatively and postoperatively for endotracheal intubation, immobilization and decreasing barotrauma, oxygen consumption, and anesthetic or sedative requirements. While some data exist in neonates and infants, the only current consensus guidelines for use of NMBs in the pediatric population comes from the United Kingdom [59]. However, this consensus only addresses use in critically ill children, and does not include neonates.
Categorized into depolarizing and nondepolarizing agents, NMBs essentially bind directly or competitively to acetylcholine receptors (AChRs) on the neuromuscular junction (NMJ) and block transmission of the electrical impulse that causes muscle contraction. A higher volume of distribution generally means a higher concentration of drug needed to reach motor endplates; however, the number and type of AChRs present change throughout development. In neonates, maturation of the NMJ occurs during the first two months of life [60]. Mature AChRs are produced only in the junctional area, but an immature type of receptor is also formed during development and in cases of denervation. These immature AChRs are located in areas outside of the junctional area, have a greater affinity for succinylcholine than nondepolarizing agents, and have sodium channels that are more easily depolarized and open for a longer period of time [61]. Clinically, this may result in variability and unpredictability of results after administration of NMBs. Older children have a higher muscle mass:fat ratio compared to infants, so will have more AChRs and require a higher drug concentration for a desired effect [60].
Succinylcholine is the only nondepolarizing agent used clinically and it is well established that larger doses are required in neonates and infants because of their increased volume of distribution [62]. Recommended doses are 2–3 mg kg–1 in neonates and infants, 1 mg kg–1 in older children, and 4 mg kg–1 for intramuscular administration. Succinylcholine is hydrolyzed by plasma cholinesterases into succinic acid and choline, so conditions like liver disease and atypical plasma cholinesterase may prolong its metabolism. Acute denervation injuries, crush injuries, and burns can result in a hypersensitivity to succinylcholine and lead to hyperkalemia, hyperkalemic dysrhythmias, or cardiac arrest. Children with undiagnosed myopathies, such as Duchenne muscular dystrophy, are at risk for malignant hyperthermia after succinylcholine administration. These and other known serious side-effects have led to the black box warning that recommends limiting use of succinylcholine to rapid sequence intubation and emergent securing of an airway in children [63].
The nondepolarizing muscle relaxants can be categorized into long-, intermediate-, and short-acting groups. With the exception of cisatracurium, they are primarily metabolized by the liver, with biliary and renal excretion. The comparative effective dose to produce 95 percent depression of twitch height (ED95) for commonly used NMBs is listed in Table 13.2.
ED95 (mcg/kg) | Onset (min) | Duration (min) | Recovery (min) | |||||||||
---|---|---|---|---|---|---|---|---|---|---|---|---|
Infant | Child | Adult | Infant | Child | Adult | Infant | Child | Adult | Infant | Child | Adult | |
Pancuronium | 66 | 93 | 67 | 2–5 | 2–4 | 3–5 | – | 24 | 22 | – | 33 | 37 |
Cisatracurium | – | 41 | 48 | – | 1–3 | 2–4 | – | 25 | 25 | – | 40 | 45 |
Vecuronium | 47 | 81 | 43 | – | 1–3 | 2–3 | – | 22 | 26 | 73 | 35 | 53 |
Rocuronium | 255 | 402 | 350 | – | 0.8–1.5 | 1–2 | – | 27 | 42 | – | 42 | 69 |
Succinylcholine | 650 | 400 | 300 | 0.5 | 0.6 | 1 | 4.6 | 5.8 | 6.0 | 7.4 | 9.7 | 10 |
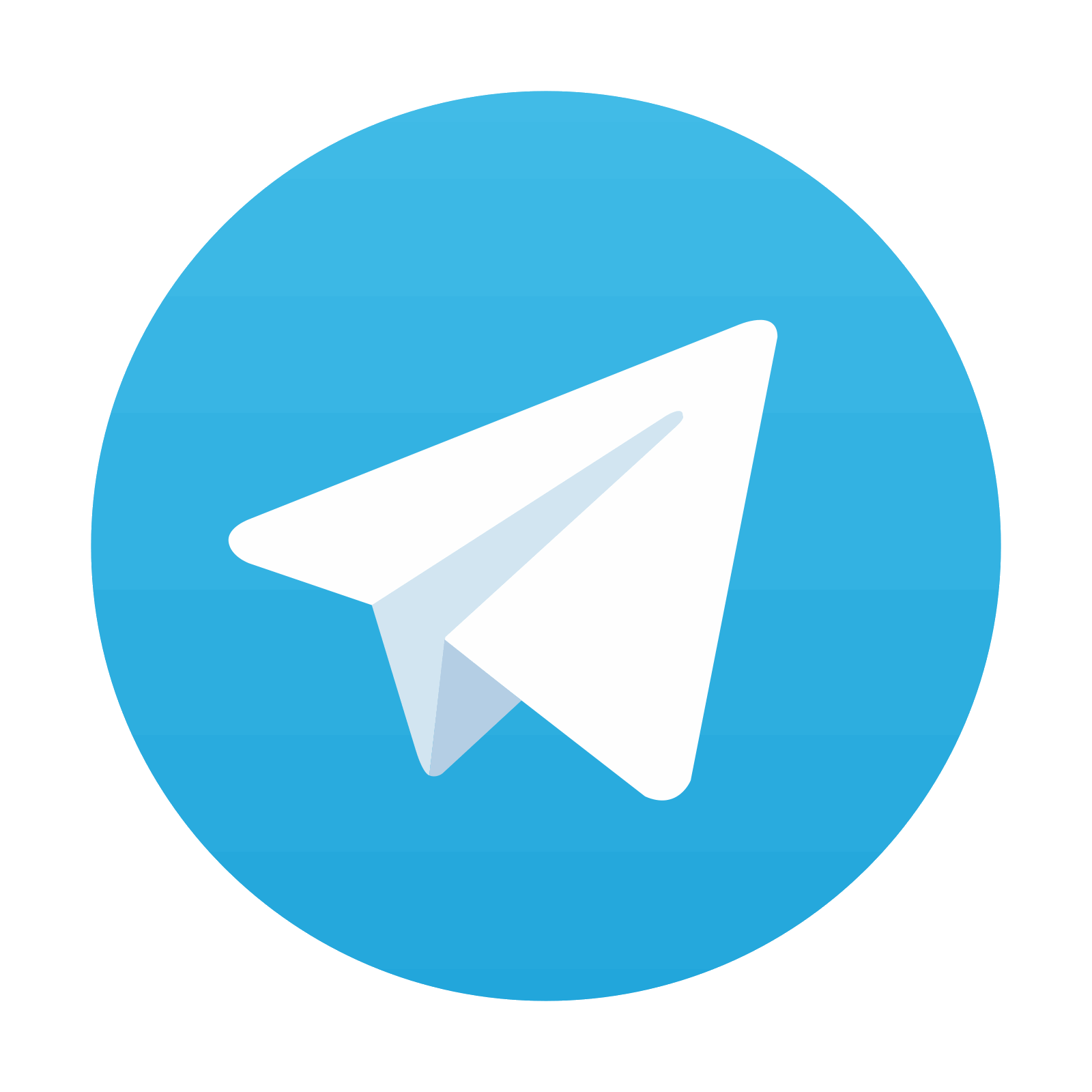
Stay updated, free articles. Join our Telegram channel
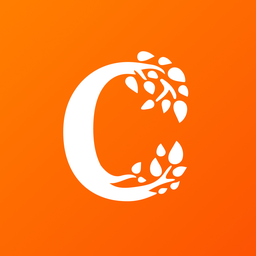
Full access? Get Clinical Tree
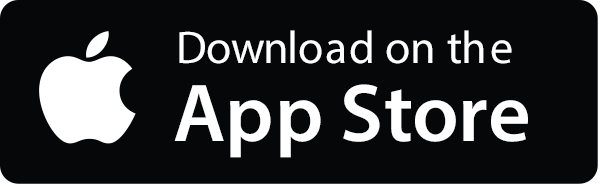
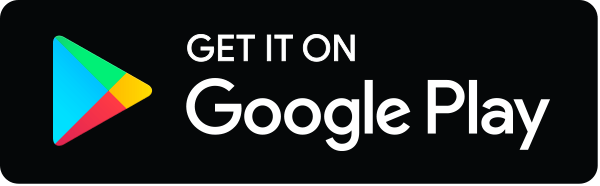
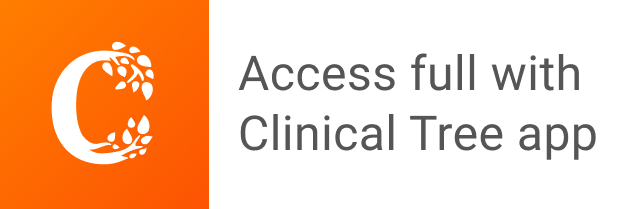